Sponsored Content by MerckFeb 1 2024Reviewed by Emily Magee
3D bioprinting is a rapidly evolving field with immense potential for creating living tissue constructs.1,2
Image Credit: Ladanifer/Shutterstock.com
Commonly, it involves a computer-assisted motorized device that deposits biocompatible materials, viable cells, growth factors, proteins, nucleic acids, drugs, and supporting components in a layer-by-layer fashion. This method allows for the precise formation of functionally and structurally biomimetic geometries that closely resemble natural tissue constructs.
In recent years, the progress in 3D bioprinting has greatly enhanced tissue and disease modeling, aiding drug development and therapeutic screening.
Generally, bioprinting relies on several main technological approaches, including extrusion, inkjet, laser-assisted, or stereolithographic methods.3 Extrusion-based bioprinting uses mechanical or pneumatic forces to dispense a bioink via a nozzle, creating a continuous filament.
Despite being relatively slow with lower resolution, this method can produce constructs from high-viscosity bioinks with reasonable cell viability.
As the most commonly used bioprinting method currently, extrusion-based bioprinting has its drawbacks, like slow and lower resolution, but it excels in working with high-viscosity bioinks.
On the other hand, inkjet bioprinting employs thermal, piezo, or acoustic forces to deposit a bioink as droplets, allowing for rapid fabrication speeds but resulting in low cell densities. It is important to note that while extrusion bioprinting can handle high-viscosity bioinks, inkjet bioprinting requires bioinks with lower viscosities.
Laser-assisted bioprinting, a nozzle-free technique, employs laser pulses to transfer bioink from the donor slide to the receiver substrate. While it allows the deposition of highly viscous and densely cellularized bioinks, it does have its limitations due to a lower cell survival rate.
Stereolithographic bioprinting utilizes precisely controlled light patterns to photo-polymerize photosensitive polymers on a vertically movable collecting platform, forming 3D constructs layer by layer. This method offers higher resolution, rapid fabrication, and increased cell viability compared to other techniques.
Despite the diverse approaches, most bioprinting processes follow a similar series of steps:
- Constructing 3D model using computer-aided design software.
- Developing/selecting a bioink, typically a combination of compatible biomaterials and viable cells based on the modality and specific tissue for bioprinting.
- Robotically programmed bioprinting with in-printing and/or post-printing physical and/or chemical crosslinking.
- Maturation of the bioprinted tissue construct. Generally, matured bioprinted tissue constructs are specifically designed for applications in tissue engineering and regenerative medicine.2
Recently, 3D bioprinting approaches have been employed to fabricate functional tissue models for disease modeling, drug development, and screening personalized therapeutics.4
This article offers a concise overview of bioink choices, accompanied by representative examples that highlight the use of 3D bioprinting in the biofabrication of tissue models (Figure 1).
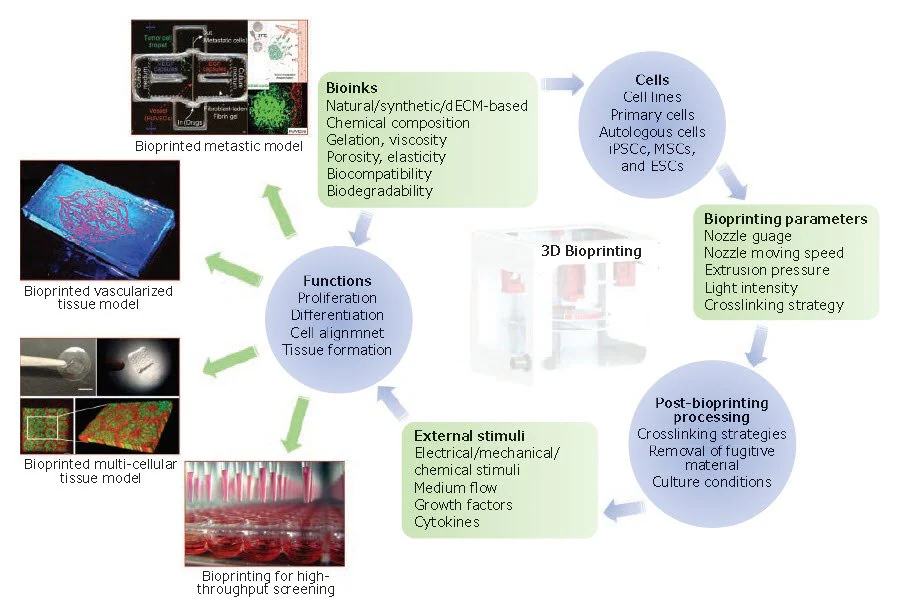
Figure 1. The essential components and applications of 3D bioprinting. Bioinks, bioprinting parameters, and post-bioprinting processing all impact viability and functionality of cells, which in turn affect subsequent cellular events, such as proliferation, differentiation, and tissue formation. hiPSCs: Human induced pluripotent stem cells, MSCs: Mesenchymal stem cells and ESCs: Embryonic stem cells.18, 27, 28 Image Credit: Merck
Bioink development and choice of biomaterials
Bioinks stand out as a vital element in 3D bioprinting. Essentially, a bioink is a hydrogel biomaterial incorporating one or more cell types, nutrients, and growth factors in varying proportions, emulating the extracellular matrix (ECM) of the tissue to support the growth of embedded cells.5
Despite their potential, designing bioinks that are biologically relevant poses a crucial challenge in the creation of 3D-bioprinted tissue constructs.
The bioink's role goes beyond providing structural, physical, and mechanical support to embedded cells. It must also furnish them with essential biological and chemical cues for cell survival, proliferation, and differentiation, crucial for tissue morphogenesis and homeostasis.
Choosing the right biomaterial (or a combination of biomaterials) for a bioink is a pivotal step in the successful bioprinting of tissues. This choice depends on various factors, such as the bioprinting modality, the targeted tissue, and any necessary post-printing processes.
In essence, an ideal bioink should:
- Exhibit good printability
- Be curable using a cell-friendly method
- Possess suitable mechanical strength to uphold the structural integrity of the targeted tissue
- Be biocompatible and biodegradable without triggering toxicity or immunological reactions
- Replicate the in vivo microenvironment to support and enhance cellular activities, including adhesion, migration, proliferation, and differentiation of living cells (Figure 2A–B).
To date, a variety of hydrogel biomaterials have been employed in formulating bioinks for 3D bioprinting of tissues (Figure 2C).
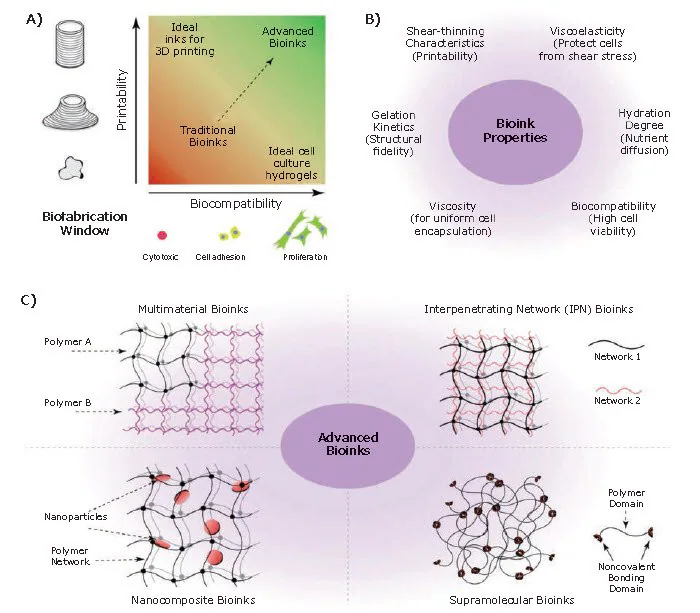
Figure 2. Bioinks for 3D bioprinting. A) Rational approach for designing bioinks requires considering both printability and biocompatibility. B) Properties of an ideal bioink. C) Classification of advanced bioinks into four major groups.5 Image Credit: Merck
This includes biomaterials derived from natural sources, such as gelatin, alginate, collagen, fibrin, silk proteins, hyaluronic acid, chitosan, and decellularized extracellular matrix (dECM), along with synthetic materials like poly(ethylene glycol) (PEG), poly(hydroxyethyl methacrylate), and polyvinyl alcohol.4
Naturally-derived biomaterials from sources like collagen offer biocompatibility, tunable degradation, and an intrinsic resemblance to native ECM, despite common drawbacks like weak mechanical strength and variability in compositions and properties among production batches. 6
On the flip side, synthetic biomaterials are highly defined and reproducible, with tunable compositions. Additionally, they are often biologically inert, being both non-toxic and non-immunogenic. The mechanical properties, degradation rate, and composition of synthetic biomaterials can be easily controlled.
Nevertheless, synthetic materials often lack sufficient sites for cell adhesion and fail to replicate the intricacy of native ECM. 7 Hence, they are frequently combined with natural biomaterials to address these limitations and engineer tissue-like microenvironments that mirror both the chemical and physical characteristics of native ECM.
For instance, 4-arm PEG-tetraacrylate (PEGTA)-gelatin methacryloyl (GelMA) and 8-arm PEG-octoacrylate (PETOA)-GelMA composite bioinks have been developed for 3D bioprinting of biomimetic vascular tissues.8,9
The addition of PEG derivatives to GelMA provides the necessary rheological and mechanical properties for bioprinting complex multilayer hollow structures.
Despite these advancements, composite hydrogels may still lack adequate biomimetic physicochemical properties for delivering tissue-specific functions. Consequently, decellularized ECM (dECM)-derived hydrogels have gained popularity as bioinks.
The decellularization process involves mechanical, chemical, and enzymatic treatments to remove cellular components, resulting in a collagenous matrix that retains many structural ECM components and soluble factors.
Studies indicate that dECM preserves the biochemical composition of the native ECM tissue, which is crucial for maintaining cell phenotypes and functionality when forming biologically relevant tissues. For instance, bioprinted renal constructs using kidney dECM-derived bioink exhibited physiologically relevant features of native renal tissue.10
Similarly, 3D bioprinting of dECM-based bioinks from pepsin-solubilized heart, adipose, and cartilage tissues demonstrated high cell viability and functionality of bioprinted rat myoblasts, human adipose-derived stem cells, and human mesenchymal stem cells in the respective tissue constructs.11
Bioprinted tissue/Disease models and their applications in probing drug effects
Tissue constructs that have been bioprinted with precisely controlled architectures serve as crucial in vitro tools for tissue and disease modeling, particularly in the field of toxicology.
These models enable the investigation of biochemical, genetic, and histological impacts of specific drugs, offering valuable information on pharmacokinetics, pharmacodynamics, and toxicity.
This section explores various applications of 3D-bioprinted tissue and disease models, focusing on the examination of tissue-specific functions, drug-metabolizing activities, and drug responses. A list of representative bioprinted tissue models employed in drug screening applications is provided in Table 1.
Table 1. Representative bioprinted tissue models for drug screening applications. Source: Merck
3D-bioprinted tissue models |
Bioprinting modality |
Bioink(s) |
Cell Type(s) |
Compounds tested |
Ref |
Cardiac tissue |
Extrusion |
Fibrinogen, gelatin, aprotinin, glycerol, hyaluronic acid |
Rat primary cardiomyocytes |
Epinephrine, carbachol |
12 |
Endothelialized cardiac tissue |
Extrusion |
Alginate, GelMA |
Neonatal rat cardiomyocytes HUVECs |
Doxorubicin |
13 |
Renal proximal tubule |
Extrusion |
Pluronic F127, thrombin; gelatin, fibrinogen, transglutaminase |
Human primary proximal tubule epithelial cells, human neonatal dermal fibroblasts |
Cyclosporine A |
15 |
Vascularized renal proximal tubule |
Extrusion |
Pluronic F127, thrombin; gelatin, fibrinogen, transglutaminase, PEO. |
Human primary proximal tubule epithelial cells, human primary glomerular microvascular endothelial cells |
Albumin, inulin, glucose, dapagliflozin |
16 |
Liver tissue |
Stereolithography |
GelMA, glycidal methacrylate-hyaluronic acid |
hiPSC-derived hepatic cells, HUVECs, human adipose-derived stem cells |
Rifampicin |
18 |
Extrusion |
NovoGel 2.0 |
Human primary parenchymal cells, HUVECs, human primary hepatic stellate cells |
Trovafloxicin, levofloxacin |
19 |
Extrusion |
GelMA |
HepG2/C3A human hepatocellular carcinoma cell line |
Acetaminophen |
21 |
Intestinal tissue |
Extrusion |
NovoGel |
Human primary intestinal epithelial cells, human primary intestinal myofibroblasts, Caco-2 human intestinal epithelial cell line |
Indomethacin, rhodamine 123, lucifer yellow, mitoxantrone, digoxin, propranolol, topotecan |
22 |
Tumor models |
Glioma |
Extrusion |
Alginate, gelatin, fibrinogen |
SU3 human glioma stem cell line, U87 human glioma cell line |
Temozolomide |
23 |
Cervical cancer |
Extrusion |
Alginate, gelatin, fibrinogen |
HeLa human cervical epithelial carcinoma cell line |
Paclitaxel |
24 |
Glioma |
Extrusion |
GelMA, gelatin |
RAW264.7 mouse macrophage cell line, GL261 mouse glioblastoma cells |
Carmustine, AS1517499, BLZ945 |
25 |
Ovarian cancer |
Droplet |
Matrigel |
OVCAR-5 human ovarian cancer cell line, MRC-5 human lung fibroblasts |
|
26 |
Vascularized lung cancer |
Droplet |
PLGA; fibrin |
A549 human lung cancer cell line, HUVECs |
Immunotoxin EGF4KDEL, CD22KDEL |
27 |
Cardiac tissue models
Bioprinting can potentially be used to produce physiologically relevant 3D contractile cardiac tissues for drug testing purposes.
For instance, rat primary cardiomyocytes, encapsulated in a fibrin-based bioink containing fibrinogen, gelatin, aprotinin, glycerol, and hyaluronic acid, were bioprinted to form cardiac tissue constructs exhibiting spontaneous and synchronous contractions during in vitro culture.12
These constructs were assessed for physiological responses to known cardiotoxic drugs such as epinephrine and carbachol. Notably, epinephrine (200 nM) increased the beating frequency from 80 to 110 beats per minute, while carbachol (10 μM) decreased the beating frequency to 40 beats per minute.
Highlighting the crucial role of blood vessels in transporting nutrients, oxygen, and drugs within tissues, including the heart, a recent study showcased the fabrication of 3D endothelialized microfibrous scaffolds.
This was achieved through extrusion bioprinting, using a bioink blend of alginate/GelMA loaded with human umbilical vein endothelial cells (HUVECs) (Figure 3A–B).
Subsequently, these scaffolds were seeded with neonatal rat cardiomyocytes or iPSC-derived cardiomyocytes, resulting in the creation of endothelialized myocardial tissues capable of spontaneous and synchronous contractions (Figure 3C).13
Subsequently, this tissue model was incorporated into a microfluidic bioreactor, enabling the examination of the toxicity of the anti-cancer drug doxorubicin in a dose- and time-dependent manner.
Following exposure to doxorubicin for six days, the beating rate of cardiomyocytes decreased, accompanied by a simultaneous reduction in the secretion of von Willebrand factor (vWF) by the HUVECs (Figure 3D–E).
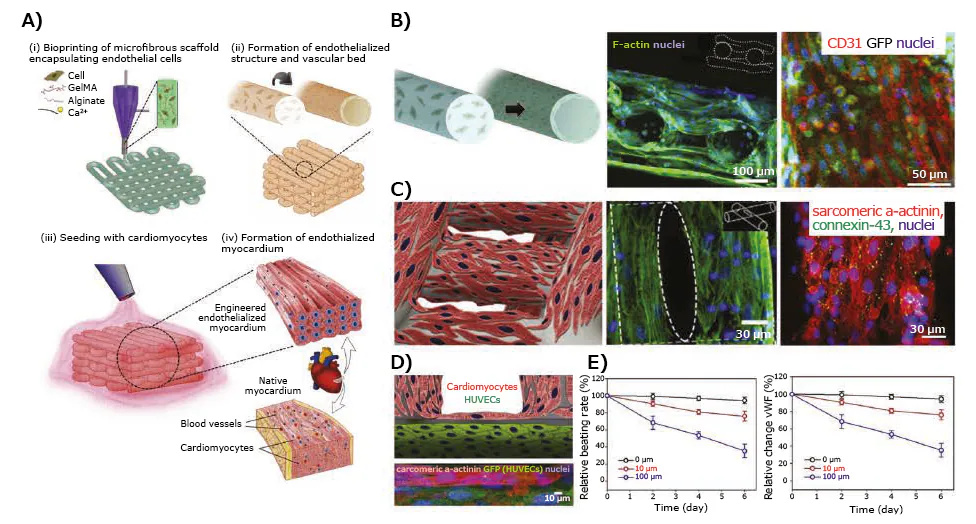
Figure 3. Application of 3D-bioprinted tissue models in drug testing — 3D-bioprinted endothelialized myocardium model. A) Schematics showing the procedure of fabricating endothelialized myocardium tissue model. B) Schematic showing the assembly of HUVECs in the bioprinted microfibers into a confluent layer of endothelium on the peripheries, as well as confocal fluorescence images showing the cross-sectional view of a three-layer scaffold, and tight junction formation between the HUVECs. C) Schematic showing a scaffold seeded with neonatal rat cardiomyocytes, F-actin staining showing the distribution of cardiomyocytes on the surface of the microfibers, and immunofluorescence staining of sarcomeric α-actinin and connexin-43 expressions. D) Schematic and high-resolution confocal fluorescence micrograph showing an endothelialized myocardial tissue. E) Relative beating of the endothelialized myocardial tissues and the levels of vWF expression by the endothelial cells, upon treatment with different dosages of doxorubicin.13 Image Credit: Merck
Kidney tissue models
Human kidneys filter approximately 180 L of plasma daily, reabsorbing water and solutes through renal tubules while eliminating waste from the blood,14 rendering them vulnerable to damage by drugs and toxins.
Bioprinting presents a promising approach for creating kidney tissues or components, like renal tubules, capable of performing key kidney functions such as filtration, reabsorption, and secretion.
Homan et al. utilized extrusion bioprinting to bioprint renal proximal tubules. They employed a sacrificial bioink made of Pluronic F127 and thrombin, initially printing it on a gelatin-fibrinogen-transglutaminase ECM. Subsequently, the Pluronic F127 was removed, resulting in the formation of a hollow tubule within the crosslinked ECM (Figure 4A–B).15
Proximal tubule epithelial cells were then seeded within the tubule, growing to maturity under continuous medium perfusion through the lumen (Figure 4C). These perfusable proximal tubules exhibited an epithelial-like morphology, disrupted in a dose-dependent manner when treated with cyclosporine A (Figure 4D).
The same research group later reported16 the bioprinting of a 3D vascularized proximal tubule model. This model consisted of two adjacent proximal tubules and vascular conduits embedded in an ECM, using their original ECM and fugitive bioink with slight modifications (Figure 4E).
Proximal and vascular channels were seeded with proximal tubule epithelial cells and glomerular microvascular endothelial cells, respectively (Figure 4F). The study of bio-macromolecule uptake using fluorescently labeled albumin and inulin revealed selective reabsorption of albumin.
Epithelium-endothelium crosstalk was investigated by circulating a high-glucose (400 mg glucose/dL) medium with or without dapagliflozin and normal glucose (100 mg glucose/dL) medium through the proximal tubule, monitoring both glucose reabsorption and endothelial cell dysfunction.
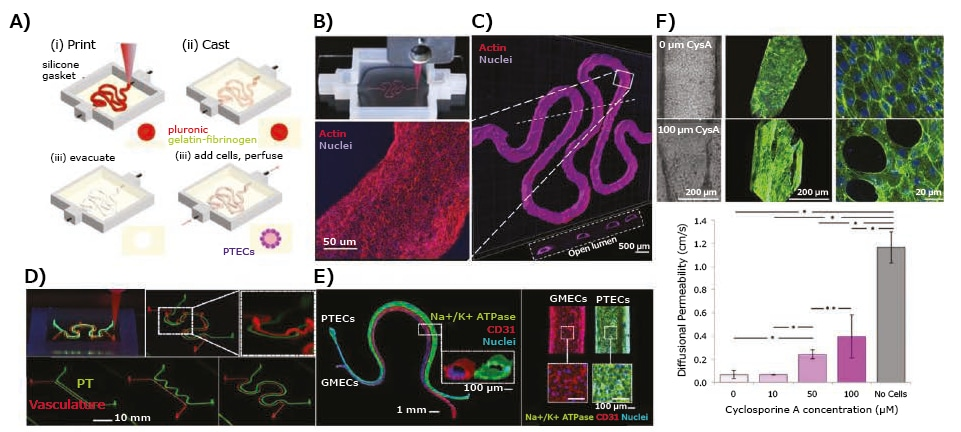
Figure 4. Application of 3D-bioprinted tissue models in drug testing — 3D-bioprinted convoluted renal proximal tubule model. A) Schematics of different steps in the fabrication of 3D renal proximal tubule. B) Photograph showing the bioprinting process of a proximal tubule (Pluronic F127 fugitive template). C) Confocal projection and 3D rendering images of the bioprinted convoluted proximal tubule populated with a confluent layer of proximal tubule epithelial cells. D) Cyclosporine A-induced disruption of the epithelial barrier function by quantifying the diffusional permeability of fluorescein isothiocyanate (FITC)-dextran (70 kDa). E) Simple and complex bioprinted vascularized proximal tubule (3D VasPT) models. F) Confocal images of the 3D VasPT containing epithelial cells in the proximal tubule and endothelial cells in the vessel. Reproduced under the Creative Commons Attribution License 4.0.15,16 Image Credit: Merck
Liver tissue models
Hepatotoxicity remains a primary cause of late-stage drug failures,17 emphasizing the importance of liver drug toxicity studies in drug development. In this context, 3D bioprinting has been utilized to create liver tissue models that faithfully replicate drug metabolism, as well as glucose and lipid metabolisms.
For instance, digital light processing-based stereolithographic bioprinting was employed to construct a 3D hepatic lobule model.
This model featured patterned human induced pluripotent stem cell (hiPSC)-derived hepatic cells, HUVECs, and adipose-derived stem cells in a physiologically relevant architecture using GelMA and glycidal methacrylate-hyaluronic acid.18
The study investigated the expression of hepatic marker genes and enzymes involved in drug metabolism in hiPSC-derived hepatic progenitor cells (hiPSC-HPCs) within the bioprinted 3D hepatic model.
Notably, among the five cytochromes P450 (CYP1A2, CYP2B6, CYP2C9, CYP2C19, and CYP3A4), CYP3A4 showed significantly higher expressions in hiPSC-HPCs. Since approximately half of today's drugs are estimated to be metabolized by CYP3A4,18 this model proves promising.
The study also assessed the impact of rifampicin, an antibiotic with potential hepatotoxicity, revealing significant increases in the expressions of CYP3A4, CYP2C9, and CYP2C19 in hiPSCHPCs within the 3D-bioprinted hepatic model compared to untreated controls.
In another study by Nguyen et al.,19 a liver tissue model was fabricated using the micro extrusion method, employing primary human parenchymal cells (100% hepatocyte cellular paste, generated via compaction) and non-parenchymal cells (HUVECs and hepatic stellate cells in NovoGel hydrogel).
Their responses to the known hepatotoxicant trovafloxacin were studied in comparison to levofloxacin, revealing that trovafloxacin induced significant toxicity at clinically relevant doses (≤ 4 μM) in a dose-dependent manner.
Similarly, an extrusion-bioprinted liver model using HepG2 cell-laden Matrigel was employed in a microfluidic system to analyze the metabolism of amifostine, an anti-radiation prodrug.20 Furthermore, 3D bioprinting of human HepG2/C3A spheroids was achieved within the GelMA bioink directly in a bioreactor (Figure 5A–B).21
The liver-on-a-chip platforms with bioprinted hepatic spheroids were cultured under medium perfusion and analyzed for albumin, alpha-1 antitrypsin (A1AT), transferrin, and ceruloplasmin secretions, as well as for expressions of cytokeratin 18, multi-drug resistance-associated protein 2 (MRP-2), and tight junction protein ZO-1 in hepatocytes within the bioprinted constructs (Figure 5C).
The toxicity of acetaminophen (APAP) was further evaluated (Figure 5D), demonstrating the application of the model for drug toxicity testing.
Intestinal tissue models
The intestine stands out as one of the primary organs for drug reabsorption. Recently, a bi-layered intestinal tissue model was crafted through extrusion bioprinting.
An interstitial layer was generated using adult human intestinal myofibroblasts, while an epithelial layer employed adult human intestinal epithelial cells suspended in a thermo-responsive NovoGel.22
The resulting intestinal tissue constructs exhibited a polarized epithelium expressing tight junction proteins such as E-cadherin, ZO-1, and functional CYP450 enzymes. Permeability studies were conducted using Lucifer yellow, mitoxantrone, digoxin, propranolol, and topotecan.
Additionally, the toxicity of indomethacin, a nonsteroidal anti-inflammatory drug, was investigated, revealing a dose-dependent decrease in barrier function in the bioprinted intestinal tissue.
Tumor models
Beyond normal tissues, bioprinting has demonstrated promise in crafting tumor tissue models that more accurately replicate the native tumor microenvironment. This is crucial for understanding tumor cell proliferation, metastatic dissemination, and responses to pharmaceutical agents.
An extrusion-based 3D bioprinted glioma model was also established using a glioma stem cell-laden porous alginate/gelatin/fibrinogen bioink.23 Drug-sensitivity studies with this glioma model revealed increased resistance to temozolomide compared to monolayer cultures at concentrations of 400–1600 μg mL-1.
In a recent development, a two-step extrusion bioprinting of mini-brains was reported. The larger brain tissue was initially bioprinted with an empty cavity using mouse macrophage cells, and then the cavity was filled with mouse glioblastoma cells. Both cell types were encapsulated in the GelMA/gelatin blend bioink.24
Glioblastoma cells actively recruited macrophages, polarizing them into a glioblastoma-associated macrophage-specific phenotype.
The study investigated the effects of carmustine, a common chemotherapy for glioblastoma, and two immunomodulatory drugs, AS1517499 (a Stat6 inhibitor) and BLZ945 (an inhibitor of colony-stimulating factor 1 receptor (Csf-1r)).
A 3D cervical cancer tumor model was bioprinted using HeLa cells encapsulated in gelatin/alginate/fibrinogen, revealing increased chemoresistance to paclitaxel compared to planar cultures.25
In another study, a 3D ovarian cancer model was generated by microvalve cell deposition (i.e., inkjet bioprinting) using OVCAR-5 human ovarian cancer cells and MRC-5 fibroblasts micro-patterned on Matrigel.26
OVCAR-5 and MRC-5 cells were simultaneously ejected using dual ejector heads in a spatially controlled microenvironment, enabling a high-throughput and reproducible process.
OVCAR-5 cells overlaid on Matrigel spontaneously formed multicellular acini ranging in size from ~100 to 500 μm², exhibiting increasing heterogeneity over the 15-day culture period.
In a separate study, Meng et al.27 reported a 3D-bioprinted vascularized tumor model designed to mimic metastatic dissemination. This model integrated lung tumor cells (A549 cells), HUVECs-lined vascular conduits, and biochemical signals from 3D-bioprinted core/shell capsules.
The core consisted of growth factor-loaded GelMA hydrogel, surrounded by gold nanorods-functionalized poly(lactic-co-glycolic acid) (PLGA) films as shells, while a fibroblast-laden fibrin hydrogel matrix served as the primary component of the tumor stroma.
The study investigated tumor cell invasion into the surrounding matrix and intravasation into the vasculature using gradients of epidermal growth factor and vascular endothelial growth factor dynamically released from the capsules.
Additionally, the potency and targeting of two immunotoxin ligand-directed toxins, EGF4KDEL and CD22KDEL, were studied.
Conclusions
The utilization of 3D-bioprinted tissue models for in vitro drug testing has progressed significantly in recent years, offering the potential for enhanced reproducibility and reduced costs in drug discovery and development through automated bioprinting operations.
Additionally, these bioprinted tissue models hold promise for diminishing the reliance on animal testing in both academic labs and pharmaceutical companies.
Despite these advancements, several challenges persist, including the need for increased speed and resolution, the availability of tissue and/or patient-specific cells, and the requirement for proper vascularization of the tissue models.
The currently limited selection of biomaterials underscores the urgent need for innovative bioink formulations. These formulations are crucial to improving the fabrication of functional tissue constructs and facilitating their application in drug testing.
References
- Stanton MM, Samitier J, Sánchez S. Bioprinting of 3D hydrogels. Lab Chip. 15(15):3111-3115. https://doi.org/10.1039/c5lc90069g
- Murphy SV, Atala A. 2014. 3D bioprinting of tissues and organs. Nat Biotechnol. 32(8):773-785. https://doi.org/10.1038/nbt.2958
- Heinrich MA, Liu W, Jimenez A, Yang J, Akpek A, Liu X, Pi Q, Mu X, Hu N, Schiffelers RM, et al. 3D Bioprinting: from Benches to Translational Applications. Small.1805510. https://doi.org/10.1002/smll.201805510
- Ma X, Liu J, Zhu W, Tang M, Lawrence N, Yu C, Gou M, Chen S. 2018. 3D bioprinting of functional tissue models for personalized drug screening and in vitro disease modeling. Advanced Drug Delivery Reviews. 132235-251. https://doi.org/10.1016/j.addr.2018.06.011
- Chimene D, Lennox KK, Kaunas RR, Gaharwar AK. 2016. Advanced Bioinks for 3D Printing: A Materials Science Perspective. Ann Biomed Eng. 44(6):2090-2102. https://doi.org/10.1007/s10439-016-1638-y
- Keane TJ, Badylak SF. 2014. Biomaterials for tissue engineering applications. Seminars in Pediatric Surgery. 23(3):112-118. https://doi.org/10.1053/j.sempedsurg.2014.06.010
- Zhu J. 2010. Bioactive modification of poly(ethylene glycol) hydrogels for tissue engineering. Biomaterials. 31(17):4639-4656. https://doi.org/10.1016/j.biomaterials.2010.02.044
- Jia W, Gungor-Ozkerim PS, Zhang YS, Yue K, Zhu K, Liu W, Pi Q, Byambaa B, Dokmeci MR, Shin SR, et al. 2016. Direct 3D bioprinting of perfusable vascular constructs using a blend bioink. Biomaterials. 10658-68. https://doi.org/10.1016/j.biomaterials.2016.07.038
- Pi Q, Maharjan S, Yan X, Liu X, Singh B, van Genderen AM, Robledo-Padilla F, Parra-Saldivar R, Hu N, Jia W, et al. 2018. Digitally Tunable Microfluidic Bioprinting of Multilayered Cannular Tissues. Adv. Mater.. 30(43):1706913. https://doi.org/10.1002/adma.201706913
- Ali M, PR AK, Yoo JJ, Zahran F, Atala A, Lee SJ. 2019. A Photo?Crosslinkable Kidney ECM?Derived Bioink Accelerates Renal Tissue Formation. Adv. Healthcare Mater.. 8(7):1800992. https://doi.org/10.1002/adhm.201800992
- Pati F, Jang J, Ha D, Won Kim S, Rhie J, Shim J, Kim D, Cho D. 2014. Printing three-dimensional tissue analogues with decellularized extracellular matrix bioink. Nat Commun. 5(1): https://doi.org/10.1038/ncomms4935
- Wang Z, Lee SJ, Cheng H, Yoo JJ, Atala A. 2018. 3D bioprinted functional and contractile cardiac tissue constructs. Acta Biomaterialia. 7048-56. https://doi.org/10.1016/j.actbio.2018.02.007
- Zhang YS, Arneri A, Bersini S, Shin S, Zhu K, Goli-Malekabadi Z, Aleman J, Colosi C, Busignani F, Dell'Erba V, et al. 2016. Bioprinting 3D microfibrous scaffolds for engineering endothelialized myocardium and heart-on-a-chip. Biomaterials. 11045-59. https://doi.org/10.1016/j.biomaterials.2016.09.003
- Lote CJ. 2012. Principles of Renal Physiology. https://doi.org/10.1007/978-1-4614-3785-7
- Homan KA, Kolesky DB, Skylar-Scott MA, Herrmann J, Obuobi H, Moisan A, Lewis JA. 2016. Bioprinting of 3D Convoluted Renal Proximal Tubules on Perfusable Chips. Sci Rep. 6(1): https://doi.org/10.1038/srep34845
- Lin NYC, Homan KA, Robinson SS, Kolesky DB, Duarte N, Moisan A, Lewis JA. 2019. Renal reabsorption in 3D vascularized proximal tubule models. Proc Natl Acad Sci USA. 116(12):5399-5404. https://doi.org/10.1073/pnas.1815208116
- Kullak-Ublick GA, Andrade RJ, Merz M, End P, Benesic A, Gerbes AL, Aithal GP. 2017. Drug-induced liver injury: recent advances in diagnosis and risk assessment. Gut. 66(6):1154-1164. https://doi.org/10.1136/gutjnl-2016-313369
- Ma X, Qu X, Zhu W, Li Y, Yuan S, Zhang H, Liu J, Wang P, Lai CSE, Zanella F, et al. 2016. Deterministically patterned biomimetic human iPSC-derived hepatic model via rapid 3D bioprinting. Proc Natl Acad Sci USA. 113(8):2206-2211. https://doi.org/10.1073/pnas.1524510113
- Nguyen DG, Funk J, Robbins JB, Crogan-Grundy C, Presnell SC, Singer T, Roth AB. Bioprinted 3D Primary Liver Tissues Allow Assessment of Organ-Level Response to Clinical Drug Induced Toxicity In Vitro. PLoS ONE. 11(7):e0158674. https://doi.org/10.1371/journal.pone.0158674
- Snyder JE, Hamid Q, Wang C, Chang R, Emami K, Wu H, Sun W. 2011. Bioprinting cell-laden matrigel for radioprotection study of liver by pro-drug conversion in a dual-tissue microfluidic chip. Biofabrication. 3(3):034112. https://doi.org/10.1088/1758-5082/3/3/034112
- Bhise NS, Manoharan V, Massa S, Tamayol A, Ghaderi M, Miscuglio M, Lang Q, Shrike Zhang Y, Shin SR, Calzone G, et al. A liver-on-a-chip platform with bioprinted hepatic spheroids. Biofabrication. 8(1):014101. https://doi.org/10.1088/1758-5090/8/1/014101
- Madden LR, Nguyen TV, Garcia-Mojica S, Shah V, Le AV, Peier A, Visconti R, Parker EM, Presnell SC, Nguyen DG, et al. 2018. Bioprinted 3D Primary Human Intestinal Tissues Model Aspects of Native Physiology and ADME/Tox Functions. iScience. 2156-167. https://doi.org/10.1016/j.isci.2018.03.015
- Dai X, Ma C, Lan Q, Xu T. 3D bioprinted glioma stem cells for brain tumor model and applications of drug susceptibility. Biofabrication. 8(4):045005. https://doi.org/10.1088/1758-5090/8/4/045005
- Zhao Y, Yao R, Ouyang L, Ding H, Zhang T, Zhang K, Cheng S, Sun W. Three-dimensional printing of Hela cells for cervical tumor model in vitro. Biofabrication. 6(3):035001. https://doi.org/10.1088/1758-5082/6/3/035001
- Heinrich MA, Bansal R, Lammers T, Zhang YS, Michel Schiffelers R, Prakash J. 2019. 3D?Bioprinted Mini?Brain: A Glioblastoma Model to Study Cellular Interactions and Therapeutics. Adv. Mater.. 31(14):1806590. https://doi.org/10.1002/adma.201806590
- Xu F, Celli J, Rizvi I, Moon S, Hasan T, Demirci U. 2011. A three-dimensional in vitro ovarian cancer coculture model using a high-throughput cell patterning platform. Biotechnology Journal. 6(2):204-212. https://doi.org/10.1002/biot.201000340
- Meng F, Meyer CM, Joung D, Vallera DA, McAlpine MC, Panoskaltsis-Mortari A. 2019. 3D Bioprinted In Vitro Metastatic Models via Reconstruction of Tumor Microenvironments. Adv. Mater.. 31(10):1806899. https://doi.org/10.1002/adma.201806899
- Richards D, Jia J, Yost M, Markwald R, Mei Y. 2017. 3D Bioprinting for Vascularized Tissue Fabrication. Ann Biomed Eng. 45(1):132-147. https://doi.org/10.1007/s10439-016-1653-z
About Merck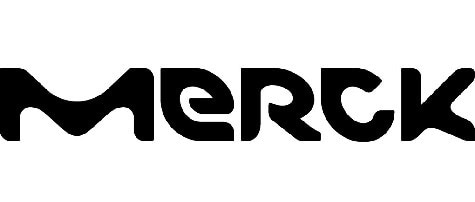
Our pursuit is progress for people everywhere. That's why we take a closer look at things, ask questions, and think ahead.
We've been around for more than 350 years, yet our majority owners are still the descendants of Friedrich Jacob Merck, the man who founded our company in Darmstadt, Germany in 1668.
From advancing gene-editing technologies and discovering unique ways to treat the most challenging diseases to enabling the intelligence of devices – the company is everywhere.
We are Merck. The only exceptions are the United States and Canada. Here we operate as EMD Serono in the Biopharma business, as MilliporeSigma in the Life Science business, and as EMD Performance Materials in the materials business.
Our life science business
We provide infinite solutions to solve the toughest problems in life science in collaboration with the global scientific community. Our tools, services, and digital platforms empower scientists and engineers at every stage, helping deliver breakthrough therapies more quickly.
Focus areas
With our three business units, we are a leading worldwide supplier of tools, high-grade chemicals, and equipment for academic labs, biotech, and biopharmaceutical manufacturers, as well as the industrial sector.
- Research Solutions provides our academic customers with the chemicals and tools needed to make scientific discovery easier and faster.
- Process Solutions provides drug manufacturers with process development expertise and technologies, such as continuous bioprocessing.
- Applied Solutions offers both testing kits and services to ensure that our food is safe to eat and our water is clean to drink.
Sponsored Content Policy: News-Medical.net publishes articles and related content that may be derived from sources where we have existing commercial relationships, provided such content adds value to the core editorial ethos of News-Medical.Net which is to educate and inform site visitors interested in medical research, science, medical devices and treatments.