Sponsored Content by MerckReviewed by Emily MageeJan 10 2024
Gelatin has been a subject of significant interest in the field of tissue engineering. It is derived from collagen, which is the primary component of the natural extracellular matrix (ECM).
Image Credit: kariphoto/Shutterstock.com
Gelatin interacts with cells through the presence of arginine-glutamine-aspartic acid (R-G-D) sequences in its protein backbone, and it is also enzymatically degradable.1
Gelatin is a cost-effective by-product of meat production,2 regarded as safe by the Food and Drug Administration (FDA) due to its extensive use in the food and pharmaceutical sectors.3
With an upper critical solution temperature (UCST) at approximately 30–35 °C, gelatin exhibits water solubility above this threshold and transforms into a hydrogel at lower temperatures.4–6
This temperature-driven shift proves advantageous for extrusion-based rapid manufacturing (RM) technologies, as the shape introduced by extrusion from a heated nozzle can be secured in a cooler environment.7
Nevertheless, this characteristic results in instability under physiological or cell culture conditions.
Gelatin was initially employed solely as a temporary cell carrier, allowing simplified cell manipulation, or it underwent stabilization through various methods like coupling primary amines present in the (hydroxy)lysine and ornithine functionalities to carboxylic acids in aspartic and glutamic acid (resulting in cross-linked hydrogel network), or by cross-linking nucleophilic functionalities using gluteraldehyde.
These approaches, however, limited control over 3D structure design.3,8,9
Fortunately, there was a significant advancement in gelatin stabilization strategies in 2000 when our research group pioneered and patented gelatin-methacrylamide (Gel-MOD). This marked the introduction of the first photocrosslinkable gelatin derivative, making material processing more convenient and straightforward.6,10
Functionalization takes place via a reaction between the primary amines found in the side chains of (hydroxy)lysine and ornithine within gelatin and methacrylic anhydride. This process introduces methacrylamides.6
Since its introduction, Gel-MOD has found application in numerous biofabrication strategies and is recognized as a field standard.4,7,11–17 Initially an academic discovery, this material is now commercially available from multiple companies as a bioink for biofabrication purposes.18–20
Following this, various other photo-crosslinkable gelatin derivatives suitable for biofabrication have surfaced, categorized into chain-growth or step-growth polymerization mechanisms.
This article concentrates on photo-crosslinkable derivatives for biofabrication with high-resolution additive manufacturing applications.
Cross-linking mechanisms
Chain-growth cross-linking
The majority of crosslinkable gelatins are created using a chain growth cross-linking method. The gelatin chains carry immobilized reactive functionalities, typically (meth)acrylates or (meth)acrylamides, which undergo polymerization with one another, resulting in the formation of brief oligomer/polymer chains linking the gelatin chains.5,6,11,21,22
This approach facilitates easy material handling, involving material dissolution and the addition of a suitable photo-initiator before the cross-linking process, without the need for a cross-linking agent.
These solutions exhibit longer stability above the UCST compared to thiolene-based systems. Thiolene-based systems have a half-life ranging from 11 hours at 0 °C to only 0.2 hours at 40 °C, as discussed below.
As an example, Gel-MOD can endure 40 °C for more than 24 hours without encountering any issues, a level of stability often essential in additive manufacturing processes or cell encapsulation experiments.5,25
One drawback of chain-growth cross-linking is the creation of a more heterogeneous network, which can result in increased shrinkage during the cross-linking process.
The kinetic profile of free radical chain-growth polymerizations is usually more intricate due to diffusion limitations, chain-length concerns, and reaction-diffusion limitations to termination, resulting in a diminished degree of control over the number of reacted functional groups.26,27
The cross-linking reaction is also susceptible to oxygen inhibition, posing challenges in cell encapsulation experiments and affecting reaction reproducibility. Thus, cross-linking gelatin typically demands higher spatiotemporal energy than the thiol-ene-based step-growth hydrogels discussed later in this article.22
Step-growth cross-linking
The second category of photo-crosslinkable hydrogels utilizes a step-growth polymerization approach to introduce cross-links into the hydrogel. A step-growth mechanism occurs between two complementary reactive groups that ideally react exclusively with each other.27
The most commonly employed cross-linking method involves thiolene “photo”-click chemistry. This method enables the creation of a network by pairing any thiol with any ‘ene’ functionality, either through a light-induced, radical-mediated thiol-ene reaction or through the formation of an anionic species that leads to thiol Michael-type addition.27
The light-triggered reaction typically advances by forming a thiol-based radical, which can arise through irradiation either with or without a photo-initiator. This radical then reacts with the double bond of the ‘ene’ species.27
Generally, the reaction performs well with any non-sterically hindered ‘ene’ functionality, but the ‘ene’ functionality should not undergo competitive chain-growth homo-polymerization, such as in the case of norbornenes and vinyl ethers.27
This choice enhances control over the reaction and ensures homogeneity within the resulting network.27
To create a thiol-ene photo-crosslinkable gelatin, it must incorporate ‘ene’ functionalities, such as norbornene, vinyl esters, or allyl ethers. These functionalities can then be cross-linked using a multi-dentate, thiolated crosslinker, such as dithiothreitol.1,22–24
Alternatively, gelatin can be thiol-functionalized and then cross-linked using a multi-dentate ‘ene’ crosslinker (e.g., polyethylene glycoldiacrylate PEGDA).28,29
Thiol-ene’ photo-click’ hydrogels form more uniform networks with less shrinkage compared to chain-growth hydrogels due to the highly orthogonal nature of the reaction.30
Additionally, they are not vulnerable to oxygen inhibition and generally exhibit faster reaction rates (e.g., gel-point for Gel-NB + dithiothreitol (DTT) 2.7 s vs Gel-MOD 64.7 s).22,27,30
The reaction rate is at its peak when norbornene functionalities are employed. This is because of their “spring-loaded” behavior, which arises from the ring strain relief upon reaction, along with the rapid rate of thiol-hydrogen abstraction by the carbon-centered radical.1,24,31
The percentage of reactive functional groups can be fully controlled by adjusting the thiol-ene ratio before cross-linking.22,27,30
In general, thiol-ene systems are more suitable for cell encapsulation than chain-growth systems, as the concentration of radical species is generally at least one order of magnitude lower compared to chain-growth hydrogel systems (where more radicals need to be formed to overcome oxygen inhibition).1,30,32
Drawbacks of the step-growth method include the need for an additional multi-dentate, thiolated crosslinker in the reaction mixture, which cross-reacts with other thiols to form disulfides.26
The likelihood of disulfide formation increases over time at elevated temperatures (e.g., the half-life of DTT at pH 8.5 shifts from 11 hours at 0 °C to only 0.2 hours at 40 °C).
This significantly complicates matters, as higher temperatures are essential to maintain gelatin solubility during the bioprinting process.25 Furthermore, step-growth hydrogels generally exhibit significantly lower storage moduli than their chain-growth counterparts.1,22
Controlling mechanical properties of photo-crosslinkable gelatins
The mechanical properties of photo-crosslinkable gelatin hydrogels can be fine-tuned by modifying various parameters of the gelatin itself or during the material processing steps.
Influencing the mechanical properties by chemical modification
The final mechanical properties of the material are significantly influenced by the number of cross-linkable functionalities.4,5
For most derivatives, the degree of substitution, which is determined by the number of reacted primary amines, can be regulated by adjusting the molar ratio of the functionalizing reagent (e.g., methacrylic anhydride,4 carbic anhydride,1 and 5-norbornene-2-carboxylic acid22) to align with the number of primary amines present in the gelatin.
By converting all primary amines into cross-linkable functionalities, the mechanical characteristics can be further enhanced by modifying the carboxylic acids in the side chains of aspartic acid and glutamic acid with additional cross-linkable functionalities like 2-aminoethyl methacrylate.5
This can result in hydrogels up to five times stiffer.5 Alternatively, the mechanical features of gelatin-methacrylamide can be adjusted by covalently linking it to a biopolymer-like alginate before cross-linking.33
While this yields a less robust hydrogel blend compared to gelatin-methacrylamide, the modification enables precise control of final mechanical properties through the integration of divalent cations to physically cross-link the alginate chains.33
The protein and polysaccharide chains form a cross-linked network, producing a hydrogel that more closely imitates the ECM.33
Influencing the mechanical properties during hydrogel processing
Once the crosslinked gelatin hydrogel is synthesized, there are multiple methods to modify its mechanical properties during the processing stage. For instance, one approach involves co-crosslinking it with another photosensitive material, which can be either natural (e.g., polysaccharide) or synthetic (e.g., PEG).1,34,35
If a single material type is preferred, the mechanical properties of the ultimate hydrogel can be altered by adjusting the gelatin concentration in the hydrogel precursor solution. The higher the initial gelatin concentration, the more rigid the resulting hydrogels become.4,5,21,22
Evidence suggests that elevated gelatin concentrations (> 15 w/v%) also harm biocompatibility.21
Variations in the applied irradiation levels during cross-linking can impact the ultimate mechanical characteristics of the material.5–7,21 In general, lower doses lead to diminished cross-link densities, yielding weaker hydrogels.7,21 However, lower doses also produce more unreacted functionalities, which could potentially be cytotoxic.
In the case of chain-growth hydrogels, lower irradiation doses often decrease reproducibility due to the intricate reaction kinetics and oxygen inhibition during cross-linking.27
Additionally, when employing highly reactive thiol-ene systems, the dose’s influence is less conspicuous as the material can achieve full cross-linking at very low doses. Nevertheless, there is a clear correlation between irradiation energy and swelling degree.
For instance, in the context of two-photon polymerization, irradiation at 20 mW with a speed of 100 mm/second leads to a fully crosslinked network when applied to gelatin-NB. However, in the case of gelatin-MOD, more than 80 mW of irradiation is needed to achieve full crosslinking.5,22
When utilizing thiol-ene hydrogel systems, additional controls can be applied. For instance, adjusting the thiol-ene ratio or the applied crosslinker amount permits precise control over the number of reacted functionalities and the mechanical properties of the end product.1,22,27
As the crosslink density increases, a higher number of reactive thiols per crosslinker molecule results in stiffer hydrogels.1
This adaptability enables the production of gelatin hydrogels that can exhibit a wide range of mechanical properties.
As a result, gelatin-based materials are highly versatile and can be effectively utilized to replicate the mechanical properties of a wide range of tissues.
Gelatin processing via additive manufacturing
Gelatin hydrogels have undergone processing using various additive manufacturing techniques, encompassing both direct and indirect methods.
In direct methods, the material is applied during the additive manufacturing (AM) step,7 while indirect methods involve applying a template generated using AM to control the shape of a secondary hydrogel material.
Indirect approaches are typically used to amalgamate the mechanical properties of a stiff polymer with the desirable cell interactivity of gelatin or to introduce complex 3D structures into materials incompatible with direct AM methods.4,12,15,36
This discussion focuses on light-induced additive manufacturing techniques, particularly high-resolution methods like two-photon polymerization (2PP), often known as direct laser writing. This technique employs two-photon absorption to induce localized cross-linking, resulting in sub-micrometer spatial resolution.
By precisely directing a femtosecond laser beam into the material, the conditions for the simultaneous interaction of two photons with half the energy needed to bridge the band gap required for photo-initiator excitation can be achieved.5,37,38
This results in highly localized polymerization. Additionally, because the probability of two-photon polymerization (2PP) is high only in the small voxel determined by the applied optics and laser power, the polymerization is confined to a 3D volume element often smaller than the expected diffraction limit.
This is very different from traditional light-based additive manufacturing techniques that use single-photon (linear) absorption, where polymerization can occur throughout the entire beam path and is limited only by its penetration depth.5,39
Consequently, structures with subcellular dimensions can be produced with 2PP, making it suitable for exploring complex cell-biomaterial interactions. In 2011, the generation of gelatin-based tissue engineering scaffolds using primary adipose tissue-derived stem cells was first reported using this method.14
Since then, numerous studies have explored 2PP processing of modified gelatins, with most utilizing gelatin-methacrylamide (Gel-MOD).23,40,41
Using this gelatin derivative, successful 2PP was reported even in the presence of living cells. While the cells did not survive direct exposure to the laser during structuring, it was possible to use 2PP to trap cells within 3D microstructures.13
Cytotoxicity was not attributed to spatiotemporal irradiation by the laser or the photo-initiator but instead could be linked to the formation of cytotoxic species within the cells as a side-product of photo-initiator activation.13
This was later confirmed with a macromolecular photo-initiator based on hyaluronic acid, enabling 2PP processing combined with the encapsulation of living cells, even within the exposed areas.42
The study indicated that the previously observed cytotoxicity originated from the penetration of the low molecular weight photo-initiator through the cell membrane, resulting in photo-oxidative damage within the cell during irradiation.
Immobilizing the photo-initiator onto a macromolecule prevented cell barrier penetration, allowing 2PP in the presence of living cells.42
Despite these successful approaches, gelatin-methacrylamide does have limitations for 2PP processing. Generally, due to poor reaction kinetics and associated mechanical properties, relatively high gelatin concentrations (>15 wt%) and light doses (e.g., 330 mW at 7 mm/second scan speed) are required to cross-link the material.13,14,40,43
Most importantly, subsequent swelling of the 2PP-produced structures can compromise the high-resolution capacity of this approach.
Alternative approaches involve applying a secondary material to serve as mechanical support,35,43 either through indirect additive manufacturing (discussed below) or by initially structuring a stronger material to act as support, such as a blend of hydrophobic acrylates, followed by subsequent gelatin crosslinking.41
Alternatively, a secondary material like poly(ethylene glycol) diacrylate (PEGDA) can be utilized to form a co-network, benefiting from the superior mechanical properties of PEG and enhanced acrylate-based reaction kinetics.35
To address these limitations, a gelatin derivative was developed in which all primary amines were converted to methacrylamides (0.385 mmol/g gelatin), while additional methacrylates were introduced onto the carboxylic acids, resulting in 1 mmol of cross-linkable groups per gram gelatin.5
Consequently, a denser gelatin network was formed, demonstrating higher stiffness and minimal post-production swelling. Moreover, the reaction kinetics improved compared to conventional gelatin-methacrylamide, resulting in a broader 2PP spatiotemporal processing range.5
In addition, 2D experiments indicated comparable biocompatibility with both fibroblasts (L929) and osteoblasts (MC3T3) for Gel-MOD-AEMA and the established Gel-MOD.5
Despite the significant improvement in 2PP processing due to the introduction of additional functionalities, the cross-linking reactions still faced challenges associated with chain-growth hydrogels, as discussed earlier.
To further enhance the material processing range, 2PP experiments were conducted using thiolene photoclick hydrogels.22,23 To fully exploit the improved reactivity of thiol-ene hydrogel systems, gelatin type B was modified to include norbornene functionalities.
Gel-NB was then processed via 2PP using DTT as the thiolated crosslinker, resulting in a significantly improved spatiotemporal 2PP processing range compared to all previously reported gelatin derivatives.
Only half of the energy was required to achieve reproducible cross-linking (i.e., 20 mW at 100 mm/second for Gel-NB DS 63 vs. 40 mW at 100 mm/second for Gel-MOD-AEMA), despite a four times lower concentration of crosslinkable functionalities (i.e., 0.24 mmol/g for Gel-NB vs. 1 mmol/g for Gel-MOD-AEMA).
Elevating the laser power beyond 40 mW had no impact on the hydrogel swelling behavior, indicating that the material had already undergone complete cross-linking. This differs from Gel-MODAEMA, where an increase in laser power led to a simultaneous decrease in swelling ratios.5,22
A broader concentration range could be employed for 2PP processing with Gel-NB. Reproducible structuring was achieved for the first time below a 10 w/v% gelatin concentration (i.e., 5w/v%).22
It is worth noting that Gel-NB exhibits a notably lower swelling ratio than Gel-MOD due to the hydrophobic nature of the norbornene functionalities.22
Consequently, Gel-NB demonstrates better-computer-aided design/computer-aided manufacturing (CAD/CAM) mimicry, and the lower spatiotemporal energy required for full conversion results in stiffer gels at lower laser powers.
This allows Gel-NB to fabricate complex structures capable of supporting their weight despite minimal supporting structures.
The material can be used for micro-scaffold fabrication; studies indicate that these scaffolds can also support fibroblast population growth following seven days of cell culture.22
Conclusions
Over the past two decades, numerous photo-crosslinkable gelatins suitable for tissue engineering have emerged. Successful biofabrication strategies, coupled with desirable biocompatibility, cell interactivity, and cost-effectiveness, have led to the commercialization of several photo-crosslinkable gelatin derivatives.
Due to recent successes with thiol-ene-based systems, commercialization of these derivatives is anticipated soon.
Combining off-the-shelf materials, decreasing costs, and improved additive manufacturing technologies will likely yield high-end biofabrication breakthroughs and subsequent integration into clinical settings.
Since gelatin is already an FDA-approved material with widespread applications in the food and pharmaceutical industry, it is only a matter of time before biofabrication strategies using photo-crosslinkable gelatins become commonplace in the clinic.
Acknowledgments
Jasper Van Hoorick received a FWO-SB PhD grant from the Research Foundation Flanders (FWO, Belgium). The FWOFWF grant (a bilateral Research Foundation Flanders — Austrian Science Fund project) is acknowledged for financial support.
References
- M?noz Z, Shih H, Lin C. Gelatin hydrogels formed by orthogonal thiol?norbornene photochemistry for cell encapsulation. Biomater. Sci.. 2(8):1063-1072. https://doi.org/10.1039/c4bm00070f
- Rose J, Pacelli S, Haj A, Dua H, Hopkinson A, White L, Rose F. Gelatin-Based Materials in Ocular Tissue Engineering. Materials. 7(4):3106-3135. https://doi.org/10.3390/ma7043106
- 1975. Select Committee on GRAS Substances (SCOGS) Opinion: Gelatin, Report No. 58. [Internet]. US FDA:[updated 28 Sep 2015]. Available from: https://www.fda.gov/food/generally-recognized-safe-gras/gras-substances-scogs-database
- Van Hoorick J, Declercq H, De Muynck A, Houben A, Van Hoorebeke L, Cornelissen R, Van Erps J, Thienpont H, Dubruel P, Van Vlierberghe S. 2015. Indirect additive manufacturing as an elegant tool for the production of self-supporting low density gelatin scaffolds. J Mater Sci: Mater Med. 26(10): https://doi.org/10.1007/s10856-015-5566-4
- Van Hoorick J, Gruber P, Markovic M, Tromayer M, Van Erps J, Thienpont H, Liska R, Ovsianikov A, Dubruel P, Van Vlierberghe S. 2017. Cross-Linkable Gelatins with Superior Mechanical Properties Through Carboxylic Acid Modification: Increasing the Two-Photon Polymerization Potential. Biomacromolecules. 18(10):3260-3272. https://doi.org/10.1021/acs.biomac.7b00905
- Van Den Bulcke AI, Bogdanov B, De Rooze N, Schacht EH, Cornelissen M, Berghmans H. 2000. Structural and Rheological Properties of Methacrylamide Modified Gelatin Hydrogels. Biomacromolecules. 1(1):31-38. https://doi.org/10.1021/bm990017d
- Billiet T, Gevaert E, De Schryver T, Cornelissen M, Dubruel P. 2014. The 3D printing of gelatin methacrylamide cell-laden tissue-engineered constructs with high cell viability. Biomaterials. 35(1):49-62. https://doi.org/10.1016/j.biomaterials.2013.09.078
- Van Vlierberghe S. 2016. Cross-linking strategies for porous gelatin scaffolds. J Mater Sci. 51(9):4349-4357. https://doi.org/10.1007/s10853-016-9747-4
- Bigi A, Cojazzi G, Panzavolta S, Rubini K, Roveri N. 2001. Mechanical and thermal properties of gelatin films at different degrees of glutaraldehyde cross-linking. Biomaterials. 22(8):763-768. https://doi.org/10.1016/s0142-9612(00)00236-2
- Schacht EH, Van Den Bulcke AI, Delaey B, Draye J. 2002. Medicaments Based on Polymers Composed of Methacrylamide-Modified Gelatin. US 6458386 B1. US Patent Office.
- Klotz BJ, Gawlitta D, Rosenberg AJ, Malda J, Melchels FP. 2016. Gelatin-Methacryloyl Hydrogels: Towards Biofabrication-Based Tissue Repair. Trends in Biotechnology. 34(5):394-407. https://doi.org/10.1016/j.tibtech.2016.01.002
- Markovic M, Van Hoorick J, Hölzl K, Tromayer M, Gruber P, Nürnberger S, Dubruel P, Van Vlierberghe S, Liska R, Ovsianikov A. 2015. Hybrid Tissue Engineering Scaffolds by Combination of Three-Dimensional Printing and Cell Photoencapsulation. 6(2): https://doi.org/10.1115/1.4031466
- Ovsianikov A, Mühleder S, Torgersen J, Li Z, Qin X, Van Vlierberghe S, Dubruel P, Holnthoner W, Redl H, Liska R, et al. 2014. Laser Photofabrication of Cell-Containing Hydrogel Constructs. Langmuir. 30(13):3787-3794. https://doi.org/10.1021/la402346z
- Ovsianikov A, Deiwick A, Van Vlierberghe S, Pflaum M, Wilhelmi M, Dubruel P, Chichkov B. Laser Fabrication of 3D Gelatin Scaffolds for the Generation of Bioartificial Tissues. Materials. 4(1):288-299. https://doi.org/10.3390/ma4010288
- Van Rie J, Declercq H, Van Hoorick J, Dierick M, Van Hoorebeke L, Cornelissen R, Thienpont H, Dubruel P, Van Vlierberghe S. 2015. Cryogel-PCL combination scaffolds for bone tissue repair. J Mater Sci: Mater Med. 26(3): https://doi.org/10.1007/s10856-015-5465-8
- Loessner D, Meinert C, Kaemmerer E, Martine LC, Yue K, Levett PA, Klein TJ, Melchels FPW, Khademhosseini A, Hutmacher DW. 2016. Functionalization, preparation and use of cell-laden gelatin methacryloyl?based hydrogels as modular tissue culture platforms. Nat Protoc. 11(4):727-746. https://doi.org/10.1038/nprot.2016.037
- Yue K, Trujillo-de Santiago G, Alvarez MM, Tamayol A, Annabi N, Khademhosseini A. 2015. Synthesis, properties, and biomedical applications of gelatin methacryloyl (GelMA) hydrogels. Biomaterials. 73254-271. https://doi.org/10.1016/j.biomaterials.2015.08.045
- Hölzl K, Lin S, Tytgat L, Van Vlierberghe S, Gu L, Ovsianikov A. Bioink properties before, during and after 3D bioprinting. Biofabrication. 8(3):032002. https://doi.org/10.1088/1758-5090/8/3/032002
- “Allevi3D,” . [Internet]. Available from: https://allevi3d.com/shop/
- Gelatin methacryloyl. [Internet]. Millipore Sigma. Available from: https://www.sigmaaldrich.com/catalog/substance/gelatinmethacryloyl1234598765?lang=en®ion=US
- Billiet T, Gasse BV, Gevaert E, Cornelissen M, Martins JC, Dubruel P. 2013. Quantitative Contrasts in the Photopolymerization of Acrylamide and Methacrylamide-Functionalized Gelatin Hydrogel Building Blocks. Macromol. Biosci.. 13(11):1531-1545. https://doi.org/10.1002/mabi.201300143
- Van Hoorick J, Gruber P, Markovic M, Rollot M, Graulus G, Vagenende M, Tromayer M, Van Erps J, Thienpont H, Martins JC, et al. 2018. Highly Reactive Thiol-Norbornene Photo-Click Hydrogels: Toward Improved Processability. Macromol. Rapid Commun.. 39(14):1800181. https://doi.org/10.1002/marc.201800181
- Qin X, Torgersen J, Saf R, Mühleder S, Pucher N, Ligon SC, Holnthoner W, Redl H, Ovsianikov A, Stampfl J, et al. 2013. Three-dimensional microfabrication of protein hydrogels via two-photon-excited thiol-vinyl ester photopolymerization. J. Polym. Sci. Part A: Polym. Chem.. 51(22):4799-4810. https://doi.org/10.1002/pola.26903
- Bertlein S, Brown G, Lim KS, Jungst T, Boeck T, Blunk T, Tessmar J, Hooper GJ, Woodfield TBF, Groll J. 2017. Thiol-Ene Clickable Gelatin: A Platform Bioink for Multiple 3D Biofabrication Technologies. Adv. Mater.. 29(44):1703404. https://doi.org/10.1002/adma.201703404
- Stevens R, Stevens L, Price N. 1983. The stabilities of various thiol compounds used in protein purifications. Biochemical Education. 11(2):70. https://doi.org/10.1016/0307-4412(83)90048-1
- Pereira RF, Bártolo PJ. 2015. 3D Photo-Fabrication for Tissue Engineering and Drug Delivery. Engineering. 1(1):090-112. https://doi.org/10.15302/j-eng-2015015
- Hoyle C, Bowman C. 2010. Thiol-Ene Click Chemistry. Angewandte Chemie International Edition. 49(9):1540-1573. https://doi.org/10.1002/anie.200903924
- Vlierberghe SV, Schacht E, Dubruel P. 2011. Reversible gelatin-based hydrogels: Finetuning of material properties. European Polymer Journal. 47(5):1039-1047. https://doi.org/10.1016/j.eurpolymj.2011.02.015
- Xu K, Fu Y, Chung W, Zheng X, Cui Y, Hsu IC, Kao WJ. 2012. Thiol?ene-based biological/synthetic hybrid biomatrix for 3-D living cell culture. Acta Biomaterialia. 8(7):2504-2516. https://doi.org/10.1016/j.actbio.2012.03.049
- Lin C, Ki CS, Shih H. 2015. Thiol-norbornene photoclick hydrogels for tissue engineering applications. J. Appl. Polym. Sci.. 132(8):n/a-n/a. https://doi.org/10.1002/app.41563
- Hoyle CE, Lee TY, Roper T. 2004. Thiol-enes: Chemistry of the past with promise for the future. J. Polym. Sci. A Polym. Chem.. 42(21):5301-5338. https://doi.org/10.1002/pola.20366
- McCall JD, Anseth KS. 2012. Thiol?Ene Photopolymerizations Provide a Facile Method To Encapsulate Proteins and Maintain Their Bioactivity. Biomacromolecules. 13(8):2410-2417. https://doi.org/10.1021/bm300671s
- Graulus G, Mignon A, Van Vlierberghe S, Declercq H, Fehér K, Cornelissen M, Martins J, Dubruel P. 2015. Cross-linkable alginate-graft-gelatin copolymers for tissue engineering applications. European Polymer Journal. 72494-506. https://doi.org/10.1016/j.eurpolymj.2015.06.033
- Tytgat L, Vagenende M, Declercq H, Martins J, Thienpont H, Ottevaere H, Dubruel P, Van Vlierberghe S. 2018. Synergistic effect of ?-carrageenan and gelatin blends towards adipose tissue engineering. Carbohydrate Polymers. 1891-9. https://doi.org/10.1016/j.carbpol.2018.02.002
- Brigo L, Urciuolo A, Giulitti S, Della Giustina G, Tromayer M, Liska R, Elvassore N, Brusatin G. 2017. 3D high-resolution two-photon cross-linked hydrogel structures for biological studies. Acta Biomaterialia. 55373-384. https://doi.org/10.1016/j.actbio.2017.03.036
- Houben A, Van Hoorick J, Van Erps J, Thienpont H, Van Vlierberghe S, Dubruel P. 2017. Indirect Rapid Prototyping: Opening Up Unprecedented Opportunities in Scaffold Design and Applications. Ann Biomed Eng. 45(1):58-83. https://doi.org/10.1007/s10439-016-1610-x
- Van Hoorick J, Ottevaere H, Thienpont H, Dubruel P, Van Vlierberghe S. 2018. Polymer and Photonic Materials Towards Biomedical Breakthroughs. https://doi.org/10.1007/978-3-319-75801-5
- Qin X, Ovsianikov A, Stampfl J, Liska R. 2014. Additive manufacturing of photosensitive hydrogels for tissue engineering applications. 15(3-4): https://doi.org/10.1515/bnm-2014-0008
- Ovsianikov A, Mironov V, Stampfl J, Liska R. 2012. Engineering 3D cell-culture matrices: multiphoton processing technologies for biological and tissue engineering applications. Expert Review of Medical Devices. 9(6):613-633. https://doi.org/10.1586/erd.12.48
- Ovsianikov A, Deiwick A, Van Vlierberghe S, Dubruel P, Mo?ller L, Dra?ger G, Chichkov B. 2011. Laser Fabrication of Three-Dimensional CAD Scaffolds from Photosensitive Gelatin for Applications in Tissue Engineering. Biomacromolecules. 12(4):851-858. https://doi.org/10.1021/bm1015305
- Engelhardt S, Hoch E, Borchers K, Meyer W, Krüger H, Tovar GEM, Gillner A. 2011. Fabrication of 2D protein microstructures and 3D polymer?protein hybrid microstructures by two-photon polymerization. Biofabrication. 3(2):025003. https://doi.org/10.1088/1758-5082/3/2/025003
- Tromayer M, Gruber P, Markovic M, Rosspeintner A, Vauthey E, Redl H, Ovsianikov A, Liska R. A biocompatible macromolecular two-photon initiator based on hyaluronan. Polym. Chem.. 8(2):451-460. https://doi.org/10.1039/c6py01787h
- Engelhardt S, Hoch E, Borchers K, Meyer W, Krüger H, Tovar GEM, Gillner A. 2011. Fabrication of 2D protein microstructures and 3D polymer?protein hybrid microstructures by two-photon polymerization. Biofabrication. 3(2):025003. https://doi.org/10.1088/1758-5082/3/2/025003
About Merck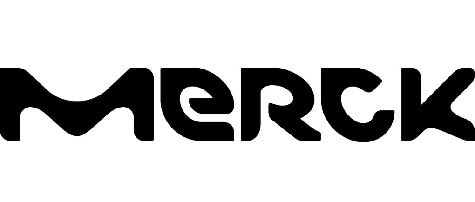
Our pursuit is progress for people everywhere. That's why we take a closer look at things, ask questions, and think ahead.
We've been around for more than 350 years, yet our majority owners are still the descendants of Friedrich Jacob Merck, the man who founded our company in Darmstadt, Germany in 1668.
From advancing gene-editing technologies and discovering unique ways to treat the most challenging diseases to enabling the intelligence of devices – the company is everywhere.
We are Merck. The only exceptions are the United States and Canada. Here we operate as EMD Serono in the Biopharma business, as MilliporeSigma in the Life Science business, and as EMD Performance Materials in the materials business.
Our life science business
We provide infinite solutions to solve the toughest problems in life science in collaboration with the global scientific community. Our tools, services, and digital platforms empower scientists and engineers at every stage, helping deliver breakthrough therapies more quickly.
Focus areas
With our three business units, we are a leading worldwide supplier of tools, high-grade chemicals, and equipment for academic labs, biotech, and biopharmaceutical manufacturers, as well as the industrial sector.
- Research Solutions provides our academic customers with the chemicals and tools needed to make scientific discovery easier and faster.
- Process Solutions provides drug manufacturers with process development expertise and technologies, such as continuous bioprocessing.
- Applied Solutions offers both testing kits and services to ensure that our food is safe to eat and our water is clean to drink.
Sponsored Content Policy: News-Medical.net publishes articles and related content that may be derived from sources where we have existing commercial relationships, provided such content adds value to the core editorial ethos of News-Medical.Net which is to educate and inform site visitors interested in medical research, science, medical devices and treatments.