Sponsored Content by MerckReviewed by Emily MageeFeb 1 2024
Over the last twenty years, polymeric materials have undergone extensive development, specifically for their use as delivery vehicles for small nucleic acids or oligonucleotides, including antisense oligonucleotides (ASO) and small interfering RNA (siRNA).1,2
Image Credit: unoL/Shutterstock.com
The application of polymeric materials in this context relies on the ability of polycations to electrostatically associate with anionic oligonucleotides in aqueous environments, forming nano complexes referred to as polyion complexes (PICs) or polyplexes.
These PICs shield oligonucleotides from enzymatic degradation and enhance their cellular uptake (or adsorptive endocytosis) by binding the positively charged PIC domain to the negatively charged cellular membrane.
Notably, chemical alterations to the constituent polycations can significantly impact the performance of PICs. This observation has driven polymer chemists and engineers to explore a range of polymers, emphasizing responsive chemistry and biocompatibility.3
This article will highlight the features of oligonucleotide-loaded PICs and the various chemical approaches that can be employed to multi-functionalize PICs, aiming for "smart" oligonucleotide delivery.
PIC formation between cligonucleotides and polycations
Poly(l-lysine) (PLys) is one of the most commonly used polycations for creating oligonucleotide-loaded PICs. This is due to its biodegradability and the easy accessibility of its pendent primary amines for chemical modifications.
Plys, with a degree of polymerization (DP) around 40, can efficiently form PICs with oligonucleotides through charge neutralization at approximately charge-equivalent mixing ratios, maintaining an [NH3+ in PLys]-to-[PO3− in oligonucleotide] ratio close to 1:1.4
Having a slight excess of PLys in the mixture can result in positively charged PICs, which is beneficial for promoting adsorptive endocytosis under standard cell culture conditions. However, positively charged nanoparticles often experience nonspecific protein adsorption, leading to the formation of larger aggregates.
To prevent aggregate formation, nonionic and hydrophilic poly(ethylene glycol) (PEG) is frequently linked to PLys (or the PIC surface).5
Typically, diblock copolymers composed of PEG and PLys (PEG-PLys) are synthesized via the ring-opening polymerization of Lys(Z) N-carboxyanhydride, using amino-terminated PEG (PEG-NH2) as a macroinitiator. This is followed by the removal of the Z group to yield PEG-PLys.7
The PEG layer sterically stabilizes PICs by reducing interactions with charged biomacromolecules, such as albumin, and minimizing interactions with neighboring PICs.6
The recent discovery regarding the PIC formation of PEG-PLys with single-stranded RNA (ssRNA) or double-stranded RNA (dsRNA) indicates a two-step assembly behavior.8 The primary step involves the creation of minimal charge-neutralized units (unit PICs) comprising RNA and PEG-PLys.
Subsequently, unit PICs undergo a secondary association, forming multimolecular assemblies progressing toward micellar or vesicular PICs (Figure 1A).
Combining ssRNA (21-mer) with PEG-PLys (molecular weight (MW) of PEG: 2,000–42,000; degree of polymerization (DP) of PLys: ~40) produced individual polyion complexes (PICs) with a hydrodynamic diameter (DH) of 10 nm at concentrations below 0.01 mg/mL.
However, at concentrations above the critical association concentration (CAC) of ~0.01 mg/mL, larger PICs with a diameter of 40 nm, termed micellar PICs, were clearly observed, as depicted in Figure 1B.
This increase in size aligns with the conventional behavior of amphiphilic macromolecules forming polymeric micelles.7
Nevertheless, there is a notable absence of secondary association (or multimolecular assembly) between dsRNA (21-mer/21-mer) and the same PEGPLys.
Unit PICs with a 10 nm diameter are produced across a broad concentration range of 0.1 to 10 mg/mL (Figure 1B).
The distinct PIC formation modes between ssRNA and dsRNA are attributed to their differing rigidity, with dsRNA's 60 nm persistence length hindering the multimolecular association of unit PICs due to decreased entropic gain associated with such assemblies.8
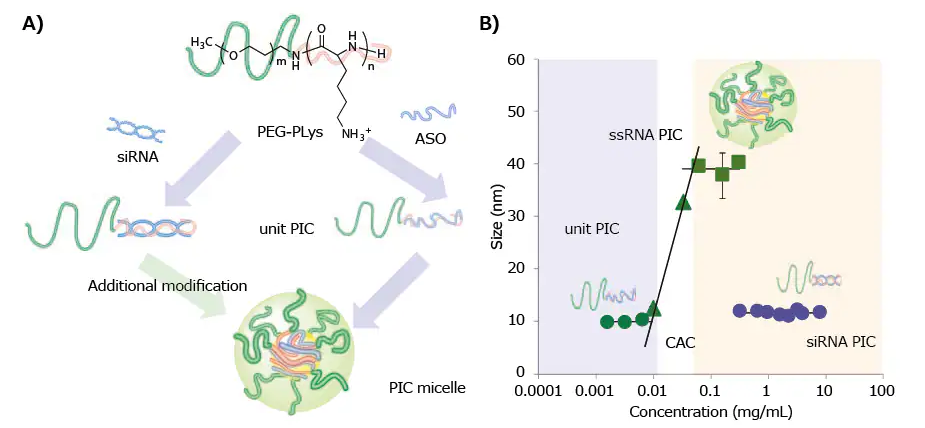
Figure 1. A) Schematic of various PIC formation behaviors between ssRNA (or ASO) and dsRNA (or siRNA) with PEG-PLys. B) Change in the hydrodynamic diameter of PICs prepared from ssRNA or dsRNA with PEG-PLys (MW of PEG: 12,000; DP of PLys: ~40) plotted as a function of concentration.8 Image Credit: Merck
Based on the formation mode of PICs, both forms of PICs, namely unit PICs and micellar PICs, are suitable for the delivery of oligonucleotides.
The potent capability of unit PICs for in vivo systemic oligonucleotide delivery was recently demonstrated.
A lengthy, two-armed PEG-PLys (PEG molecular weight: 2 × 37 kDa; PLys degree of polymerization: ~20) was devised to create 18 nm unit PICs, each consisting of a lone siRNA or ASO molecule.
These PICs displayed stability during blood circulation and effectively accumulated in murine models of fibrotic pancreatic cancer, likely due to their small size and the steric repulsion from the long, two-armed PEG.9
Unlike unit PICs, prior studies have given extensive attention to micellar PICs. Their relatively larger size (40 nm ≤ DH ≤ 100 nm) allows them to evade swift renal clearance, which has a size threshold of ~10 nm10 when administered systemically.
In this context, a crucial aspect of polymer design is the tuning of stability for the micellar states. As mentioned earlier, under diluted conditions (or at concentrations below the critical association concentration (CAC)), multimolecular PICs are prone to disintegrate into smaller fragments of unit PICs.
Therefore, to prevent micelle dissociation, additional stabilization is required. However, it is also essential that the oligonucleotide payloads are eventually released from the PICs within the target cells. This makes the reversible stabilization of PICs a significant challenge for effective systemic delivery of oligonucleotides.
Chemical modifications of PEG-PLYs for reversible stabilization of PICs
To achieve micellar PICs with reversible stability, researchers have incorporated biologically responsive linkages into polymeric materials. For instance, reduction-environment-responsive micellar PICs have been created using thiolated PEG-PLys, featuring disulfide crosslinking in the micelle core.
Disulfide-crosslinked micelles remain stable in nonreductive environments like the bloodstream but undergo destabilization within the reductive cytoplasmic compartment as the disulfide bonds are cleaved, facilitating payload release.
Thiolated PEG-PLys is synthesized by introducing thiol groups into PLys side chains using succinimidyl 3-(2-pyridyldithio)propionate (SPDP),11-13 dimethyl-3,3′-dithiobispropionimidate (DTBP),14 or 2-iminothiolane (IT).13,14 (Figure 2A)
The resulting SPDP-derived PEG-PLys (PEG-PLys(MP)), containing 10–30% thiol moieties in the PLys side chains, is mixed with single-stranded DNA (or ASO) in a buffer solution to form micellar PICs, followed by aerial oxidation to induce disulfide crosslinking in the micelle core.
The disulfide-crosslinked micelles demonstrated a diameter of 40 nm and better tolerance under non-reductive diluted conditions compared to non-crosslinked control micelles. They released the ASO payload under reductive conditions simulating the cytoplasm (1 mM glutathione).12
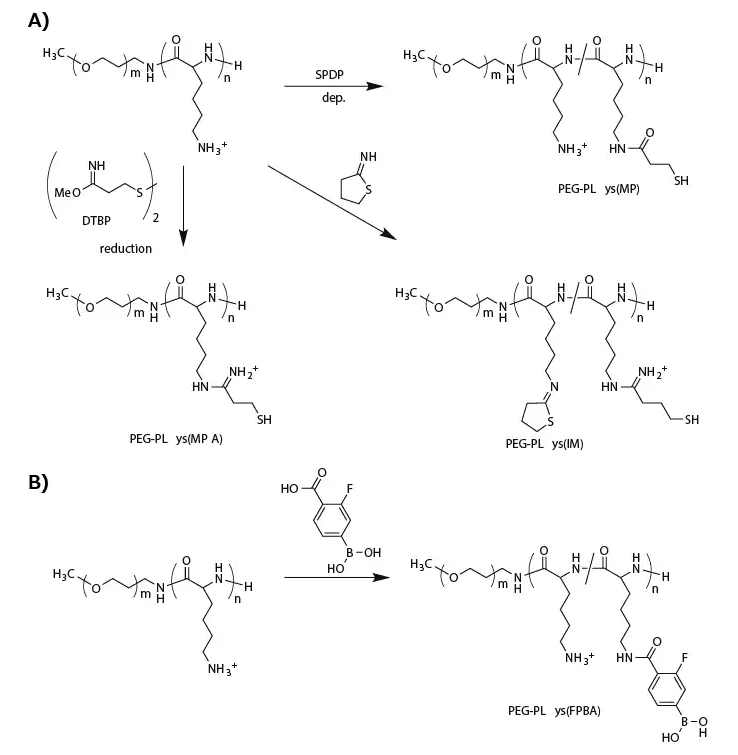
Figure 2. Functionalization of PEG-PLys for fabricating biological-environment-responsive micellar PICs. A) A series of thiolations of PEG-PLys for preparing disulfide-crosslinked micelles. B) Modification of PEG-PLys with phenylboronic acid for ATP-responsive micelle fabrication. Image Credit: Merck
A comparable crosslinking approach was explored for siRNA-loaded micellar PICs. The "rigid" siRNA-loaded micellar PICs were expected to be less stable than their ASO-loaded counterparts.
To enhance stability, more thiol groups (approximately 95% of PLys side chains) or additional stabilizing units were incorporated into the PEG-PLys. For this purpose, DTBP replaced SPDP for the thiolation of PEG-PLys, providing a positively charged amidine group and a thiol group (PEG-PLys(MPA)) (Figure 2A).
This method maintained the positively charged sites in the PLys segment even after substantial thiolation. Conversely, modification with IT introduced a closed-ring structure to the PLys segment with amidine and thiol groups (PEG-PLys(IM)) (Figure 2A).
This ring structure significantly stabilized the siRNA-loaded micellar PICs, likely due to hydrophobic interactions and/or dipole-dipole interactions. Micellar PICs prepared from PEG-PLys(IM) also exhibited a markedly prolonged blood circulation time compared to those prepared from PEG-PLys(MPA).14
Another intriguing approach involves the direct, reversible conjugation of siRNA into the PIC core. Tetravalent 3-fluorophenylboronic acid (FPBA) formed a phenylboronate ester bond with a cis-diol moiety at a physiological neutral pH.15
Consequently, FPBA-functionalized PLys side chains (Figure 2B) can covalently conjugate with the ribose ring at both 3' ends of siRNA.16 Importantly, this covalent bond is reversible and can be replaced in the presence of a high concentration of cis-diol compounds such as adenosine triphosphate (ATP).
When micellar PICs are prepared with PEG-PLys(FPBA) and siRNA, the siRNA payloads are covalently conjugated to the PLys side chains through the phenylboronate ester moiety in the PIC core, forming an siRNA-mediated crosslinked core.
Although the FPBA-crosslinked micellar PIC remained stable at low ATP concentrations (<0.3 mM, mimicking the extracellular milieu), it dissociated in the presence of high concentrations of ATP (~3 mM, mimicking the cytoplasmic condition). Thus, reversible stabilization can also be achieved using PEG-PLys with phenylboronate functionality.
Chemical modifications of PEG-PLYs for targeted oligonucleotide delivery
The PEG layer on micellar surfaces can reduce nonspecific protein adsorption while potentially hindering the adsorptive endocytosis (or cellular uptake) of associated oligonucleotide payloads.
To address this issue, functionalized micellar surfaces with ligand molecules for precise oligonucleotide delivery have been developed.17
Various ligands, such as small molecules, peptides, and antibodies, have been linked to the end of PEG to selectively bind to specific proteins or sugars overexpressed on the target cellular surface.
Importantly, the ligand-modified micellar surface facilitates multivalent binding between the micellar PIC and the target cellular surface, significantly enhancing binding affinity compared to single molecular binding.18
A cyclic RGD peptide (cRGD) was incorporated onto the micelle surface (or α-end of the PEG segment in PEG-PLys) to actively target αVβ3 and αVβ5 integrins, which are overexpressed on various cancer cells and cancer-associated endothelial cells.
The cRGD peptide was linked through thiazolidine ring formation between the formyl group in a PEG terminus and a cysteine moiety attached to a cRGD peptide (Figure 3A).19
The cRGD-conjugated micellar PIC, derived from cRGD-conjugated PEG-PLys(IM), demonstrated more efficient cellular uptake of siRNA in cultured cervical cancer cells.20
Upon systemic administration to subcutaneous-cervical-cancer-bearing mice, the cRGD-conjugated micelle significantly enhanced tumor accumulation of siRNA payloads compared to a non-cRGD-conjugated control micelle, resulting in significant antitumor activity.20,21
An antibody fragment for human tissue factor (anti-TF Fab′) was also added to the PEG terminus of micellar PICs prepared from azide-functionalized PEG-PLys(IM) through copper-free click chemistry (Figure 3A).
Notably, TF is overexpressed in various cancer and inflammatory tissues, such as pancreatic cancer and cancer-surrounding fibroblasts. Therefore, anti-TF Fab′ is suitable for cancer and cancer-associated stromal targeting.22
The anti-TF Fab′-conjugated micelle demonstrated stronger binding to TF-overexpressed pancreatic cancer cells in both monolayer and spheroid cultures.
Systemic administration of the anti-TF Fab′-conjugated siRNA-loaded micelle significantly suppressed target gene expression in a subcutaneous model of pancreatic cancer.23
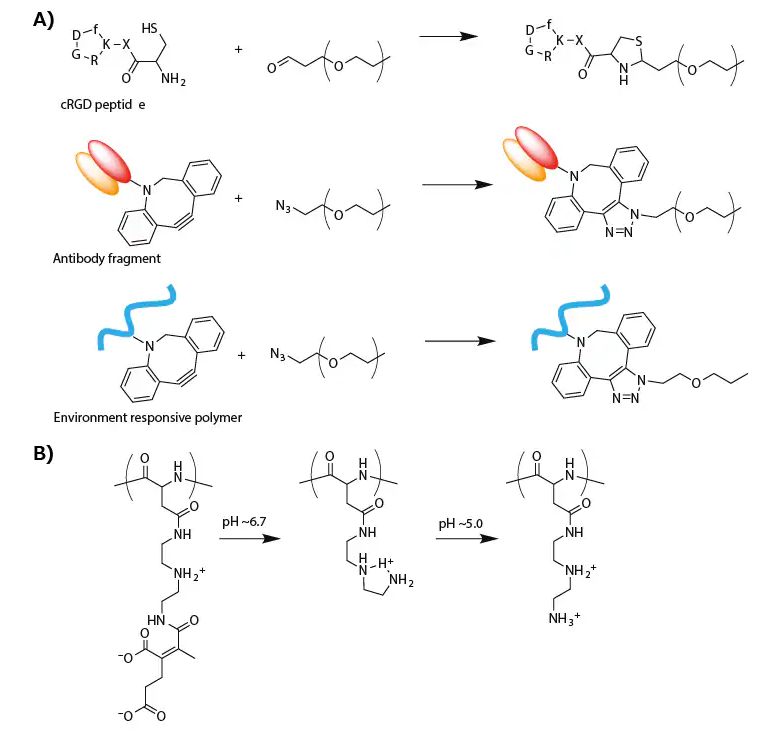
Figure 3. A) Synthesis scheme of modified PEG for targeting delivery of oligonucleotide. B) Change in the chemical structure of PAsp(DET-CDM) induced by biological pH change. Image Credit: Merck
A recent study highlighted a targeted PIC micelle linked with glucose ligands, demonstrating its ability to transcellular-ly traverse the blood–brain barrier (BBB) and reach the brain parenchyma.
This process is facilitated by the recognition of glucose-transporter 1 (GLUT1) on the brain capillary endothelial cellular surface, where GLUT1 is overexpressed.24
In the synthesis process, a hetero-bifunctionalized PEG with glucose at the α-end and a primary amine at the ω-end (Glu-PEG-NH2) was created by initiating ethylene oxide polymerization at the 6-position of glucose and aminating the other terminus.
The systemic administration of the glucose-conjugated micelle resulted in significant PIC accumulation in brain tissue (~6% dose/g brain). This approach relies on the GLUT1 recycling mechanism in brain capillary endothelial cells triggered by a glycemic control method.24
This glucose-mediated brain targeting method could find application in the systemic delivery of oligonucleotides into a diseased brain or glioblastoma.
The functionalization of PEG-PLys, responsive to the biological environment, was coupled with micellar PIC surface modification to adapt to the slightly acidic tumor microenvironment and more acidic endosomal compartment.
For this purpose, a zwitterionic polyasparamide derivative (PAsp(DET-CDM)) was synthesized from diethylenetriamine (DET) and carboxydimethyl maleate (CDM), altering the chemical structure in a stepwise manner corresponding to a biological pH change.25
Specifically, CDM moieties detached from the backbone in response to a slightly acidic tumor microenvironment (pH ≈ 6.7), while DET moieties transitioned from the monoprotonated state to the diprotonated state in an acidic late endosomal compartment (pH ≈ 5.0) (Figure 3B).
Based on this structural change, a PAsp(DET-CDM)-conjugated micellar PIC, prepared through copper-free click conjugation, exhibited a significant shift in surface potential from negative at pH 7.4 to moderately positive at pH ~6.7, facilitating interaction with the negatively charged cellular membrane in tumor tissues.
At pH ~5.0, the surface potential showed a highly positive value, destabilizing the endosomal membrane in cancer cells. In comparison to a nonconjugated control micelle, the PAsp(DET-CDM)-conjugated micelle induced efficient gene silencing in cultured lung cancer cells, particularly under a cell culture condition of pH 6.7.25
Summary
This review outlines oligonucleotide-loaded PICs created from PEG-PLys, with or without chemical modifications.
The micellar PICs showcase diverse functionalities based on their specific chemical alterations. Some of these micellar PICs have exhibited significant therapeutic effects in murine cancer models by delivering siRNAs and ASOs targeting genes related to cancer.
Despite success in vivo, clinical trials for polymeric oligonucleotide delivery systems are scarce. The primary obstacles for oligonucleotide therapeutics in clinical trials typically involve rapid renal and liver clearance.26
The stabilization of PIC micelles through chemical modifications could mitigate the pace of renal clearance. The liver tends to preferentially uptake or sequester nanoparticles with pronounced positive/negative charges, lipophilicity, or a large particle size (>100 nm).27
It is predicted that a well-thought-out design of polymeric materials for reversible stabilization and/or guiding modification, incorporating both previously reported and emerging techniques, will advance micellar delivery systems into clinical trials and, ultimately, result in therapeutic success.
References
- Kim HJ, Kim A, Miyata K, Kataoka K. 2016. Recent progress in development of siRNA delivery vehicles for cancer therapy. Advanced Drug Delivery Reviews. 10461-77. https://doi.org/10.1016/j.addr.2016.06.011
- Kaczmarek JC, Kowalski PS, Anderson DG. 2017. Advances in the delivery of RNA therapeutics: from concept to clinical reality. Genome Med. 9(1): https://doi.org/10.1186/s13073-017-0450-0
- Takemoto H, Nishiyama N. 2017. Functional polymer-based siRNA delivery carrier that recognizes site-specific biosignals. Journal of Controlled Release. 26790-99. https://doi.org/10.1016/j.jconrel.2017.09.005
- González Ferreiro M, Tillman L, Hardee G, Bodmeier R. 2001. Characterization of complexes of an antisense oligonucleotide with protamine and poly-l-lysine salts. Journal of Controlled Release. 73(2-3):381-390. https://doi.org/10.1016/s0168-3659(01)00296-6
- Kataoka K, Togawa H, Harada A, Yasugi K, Matsumoto T, Katayose S. 1996, 29, 8556–8557. Macromolecules 1996, 29, 8556–8557. [Internet]. Available from: https://doi.org/10.1021/ma961217+
- Kataoka K, Harada A, Nagasaki Y. 2001. Block copolymer micelles for drug delivery: design, characterization and biological significance. Advanced Drug Delivery Reviews. 47(1):113-131. https://doi.org/10.1016/s0169-409x(00)00124-1
- Kakizawa Y, Kataoka K. 2002. Block copolymer micelles for delivery of gene and related compounds. Advanced Drug Delivery Reviews. 54(2):203-222. https://doi.org/10.1016/s0169-409x(02)00017-0
- Hayashi K, Chaya H, Fukushima S, Watanabe S, Takemoto H, Osada K, Nishiyama N, Miyata K, Kataoka K. 2016. Influence of RNA Strand Rigidity on Polyion Complex Formation with Block Catiomers. Macromol. Rapid Commun.. 37(6):486-493. https://doi.org/10.1002/marc.201500661
- Watanabe S, Hayashi K, Toh K, Kim HJ, Liu X, Chaya H, Fukushima S, Katsushima K, Kondo Y, Uchida S, et al. 2019. In vivo rendezvous of small nucleic acid drugs with charge-matched block catiomers to target cancers. Nat Commun. 10(1): https://doi.org/10.1038/s41467-019-09856-w
- Soo Choi H, Liu W, Misra P, Tanaka E, Zimmer JP, Itty Ipe B, Bawendi MG, Frangioni JV. 2007. Renal clearance of quantum dots. Nat Biotechnol. 25(10):1165-1170. https://doi.org/10.1038/nbt1340
- Kakizawa Y, Harada A, Kataoka K. 1999. Environment-Sensitive Stabilization of Core?Shell Structured Polyion Complex Micelle by Reversible Cross-Linking of the Core through Disulfide Bond. J. Am. Chem. Soc.. 121(48):11247-11248. https://doi.org/10.1021/ja993057y
- Kakizawa Y, Harada A, Kataoka K. 2001. Glutathione-Sensitive Stabilization of Block Copolymer Micelles Composed of Antisense DNA and Thiolated Poly(ethylene glycol)-block-poly(l-lysine): A Potential Carrier for Systemic Delivery of Antisense DNA. Biomacromolecules. 2(2):491-497. https://doi.org/10.1021/bm000142l
- Miyata K, Kakizawa Y, Nishiyama N, Harada A, Yamasaki Y, Koyama H, Kataoka K. 2004. Block Catiomer Polyplexes with Regulated Densities of Charge and Disulfide Cross-Linking Directed To Enhance Gene Expression. J. Am. Chem. Soc.. 126(8):2355-2361. https://doi.org/10.1021/ja0379666
- Christie RJ, Miyata K, Matsumoto Y, Nomoto T, Menasco D, Lai TC, Pennisi M, Osada K, Fukushima S, Nishiyama N, et al. 2011. Effect of Polymer Structure on Micelles Formed between siRNA and Cationic Block Copolymer Comprising Thiols and Amidines. Biomacromolecules. 12(9):3174-3185. https://doi.org/10.1021/bm2006714
- Springsteen G, Wang B. 2002. A detailed examination of boronic acid?diol complexation. Tetrahedron. 58(26):5291-5300. https://doi.org/10.1016/s0040-4020(02)00489-1
- Naito M, Ishii T, Matsumoto A, Miyata K, Miyahara Y, Kataoka K. 2012. A Phenylboronate-Functionalized Polyion Complex Micelle for ATP-Triggered Release of siRNA. Angew. Chem. Int. Ed.. 51(43):10751-10755. https://doi.org/10.1002/anie.201203360
- Cabral H, Miyata K, Osada K, Kataoka K. 2018. Block Copolymer Micelles in Nanomedicine Applications. Chem. Rev.. 118(14):6844-6892. https://doi.org/10.1021/acs.chemrev.8b00199
- Mammen M, Choi SK, Whitesides GM. 1998, 37, 2754–2794.. Agnew Chem. Int. Ed. . [Internet]. Available from: https://onlinelibrary.wiley.com/doi/10.1002/(SICI)1521-3773(19981102)37:20%3C2754::AID-ANIE2754%3E3.0.CO;2-3
- Oba M, Fukushima S, Kanayama N, Aoyagi K, Nishiyama N, Koyama H, Kataoka K. 2007. Cyclic RGD Peptide-Conjugated Polyplex Micelles as a Targetable Gene Delivery System Directed to Cells Possessing ?v?3and ?v?5Integrins. Bioconjugate Chem.. 18(5):1415-1423. https://doi.org/10.1021/bc0700133
- Christie RJ, Matsumoto Y, Miyata K, Nomoto T, Fukushima S, Osada K, Halnaut J, Pittella F, Kim HJ, Nishiyama N, et al. 2012. Targeted Polymeric Micelles for siRNA Treatment of Experimental Cancer by Intravenous Injection. ACS Nano. 6(6):5174-5189. https://doi.org/10.1021/nn300942b
- Nishida H, Matsumoto Y, Kawana K, Christie RJ, Naito M, Kim BS, Toh K, Min HS, Yi Y, Matsumoto Y, et al. 2016. Systemic delivery of siRNA by actively targeted polyion complex micelles for silencing the E6 and E7 human papillomavirus oncogenes. Journal of Controlled Release. 23129-37. https://doi.org/10.1016/j.jconrel.2016.03.016
- van den Berg YW, Osanto S, Reitsma PH, Versteeg HH. 2012. The relationship between tissue factor and cancer progression: insights from bench and bedside. 119(4):924-932. https://doi.org/10.1182/blood-2011-06-317685
- Min HS, Kim HJ, Ahn J, Naito M, Hayashi K, Toh K, Kim BS, Matsumura Y, Kwon IC, Miyata K, et al. 2018. Tuned Density of Anti-Tissue Factor Antibody Fragment onto siRNA-Loaded Polyion Complex Micelles for Optimizing Targetability into Pancreatic Cancer Cells. Biomacromolecules. 19(6):2320-2329. https://doi.org/10.1021/acs.biomac.8b00507
- Anraku Y, Kuwahara H, Fukusato Y, Mizoguchi A, Ishii T, Nitta K, Matsumoto Y, Toh K, Miyata K, Uchida S, et al. 2017. Glycaemic control boosts glucosylated nanocarrier crossing the BBB into the brain. Nat Commun. 8(1): https://doi.org/10.1038/s41467-017-00952-3
- Tangsangasaksri M, Takemoto H, Naito M, Maeda Y, Sueyoshi D, Kim HJ, Miura Y, Ahn J, Azuma R, Nishiyama N, et al. 2016. siRNA-Loaded Polyion Complex Micelle Decorated with Charge-Conversional Polymer Tuned to Undergo Stepwise Response to Intra-Tumoral and Intra-Endosomal pHs for Exerting Enhanced RNAi Efficacy. Biomacromolecules. 17(1):246-255. https://doi.org/10.1021/acs.biomac.5b01334
- Levin AA. 2019. Treating Disease at the RNA Level with Oligonucleotides. N Engl J Med. 380(1):57-70. https://doi.org/10.1056/nejmra1705346
- Zhang Y, Poon W, Tavares AJ, McGilvray ID, Chan WC. 2016. Nanoparticle?liver interactions: Cellular uptake and hepatobiliary elimination. Journal of Controlled Release. 240332-348. https://doi.org/10.1016/j.jconrel.2016.01.020
About Merck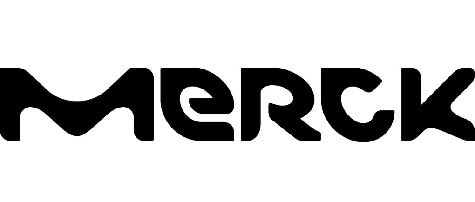
Our pursuit is progress for people everywhere. That's why we take a closer look at things, ask questions, and think ahead.
We've been around for more than 350 years, yet our majority owners are still the descendants of Friedrich Jacob Merck, the man who founded our company in Darmstadt, Germany in 1668.
From advancing gene-editing technologies and discovering unique ways to treat the most challenging diseases to enabling the intelligence of devices – the company is everywhere.
We are Merck. The only exceptions are the United States and Canada. Here we operate as EMD Serono in the Biopharma business, as MilliporeSigma in the Life Science business, and as EMD Performance Materials in the materials business.
Our life science business
We provide infinite solutions to solve the toughest problems in life science in collaboration with the global scientific community. Our tools, services, and digital platforms empower scientists and engineers at every stage, helping deliver breakthrough therapies more quickly.
Focus areas
With our three business units, we are a leading worldwide supplier of tools, high-grade chemicals, and equipment for academic labs, biotech, and biopharmaceutical manufacturers, as well as the industrial sector.
- Research Solutions provides our academic customers with the chemicals and tools needed to make scientific discovery easier and faster.
- Process Solutions provides drug manufacturers with process development expertise and technologies, such as continuous bioprocessing.
- Applied Solutions offers both testing kits and services to ensure that our food is safe to eat and our water is clean to drink.
Sponsored Content Policy: News-Medical.net publishes articles and related content that may be derived from sources where we have existing commercial relationships, provided such content adds value to the core editorial ethos of News-Medical.Net which is to educate and inform site visitors interested in medical research, science, medical devices and treatments.