Photochemical reactions begin by absorption of energy in the form of light, and involve formation of free radicals and transient excited states whose physical and chemical properties are very different to the original molecules. As an example, globally, one of the most well-known flavor defects in beer is to become skunky or ‘lightstruck’ after being exposed to UV light.
The photodecomposition of the major flavor components of hops – isohumulones, via free radical photodegradation mechanism is the reason for this ‘lightstruck flavor.’ Excited states and free radicals do not usually last long, but play important roles in significant photochemical processes such as photodegradation, polymerization, photostability, photooxidation, photocatalysis and photosynthesis.
Electron paramagnetic resonance (EPR) spectroscopy is the only analytical method which can detect species with free radicals and transient excited states in a non-invasive and direct way. It can be applied to solid, liquid, or gaseous samples over a wide range of temperatures, so this method is extremely versatile.
EPR can be employed to detect, quantify and monitor the intrinsic photogeneration of short-lived species and so is very beneficial for detecting free radicals in photochemical reactions in pharmaceuticals, polymer science, environment, etc. The intention of this article is to present a general review of the diversity of EPR applications for photochemical reactions.
Photodegradation Studies Using EPR
Light is a crucial factor when considering the stability of materials. It is important to study and evaluate it if light exposure causes unacceptable alterations in the final product or will compromise its shelf-life. For example, degradation of polymers because of light exposure results in discoloration and a reduction in the mechanical properties (toughness, elasticity, etc.).
Hindered amine light stabilizers (HALS) are added to the polymer in order to stop this decomposition. HALS prevents radical damage in the polymer by forming a HALS-based nitroxyl radical when the polymer is exposed to UV-irradiation, i.e. HALS sacrifices itself to protect the polymer molecules. EPR identifies the HALS radicals and by monitoring and quantifying the EPR signal, the effectiveness of HALS can be assessed.
This EPR application is employed successfully to establish polymer photodegradation in both industrial and academic applications for quality control and research. Ford Motor Company for example, published an EPR study in which concentrations of HALS radicals in the early stages of weather exposure supplied direct insight into the long-term weathering performance of clearcoat/basecoat automotive paint systems1.
Skin is an extremely susceptible target organ to photodegradation (photo-aging) and UV-light is the leading cause of skin disorders. These disorders include premature aging, sunburn, and photocarcinogenesis, as a result of UV-induced oxidative stress and mediated by short-lived radicals called reactive oxygen species (ROS).
So, photo-protection is critical in order to prevent undesirable effects of sun exposure and is usually accomplished by applying sunscreens and other skin care products which contain UV-filters, and antioxidants. Their radical scavenging activity can be assessed by EPR spectroscopy in addition to screening of their safety and efficacy.
Usually, the product is tested under accelerated conditions (UV-irradiation) to heighten the rate of photodegradation and the data gathered from these stability studies (stress testing) can be transferred to real time stability data. The effectiveness of antioxidants in skin care products relies on their penetration kinetics, photostability, and reactivity with non-radical components.
As an example, when three commonly utilized antioxidants (butylhydroxytoluene, green tea, and vitamin E) were examined via accelerated UV-irradiation for their effectiveness in a skin care product, the EPR data exhibited an almost ~90% reduction of the radical yield in the case of vitamin E followed by ~60% and ~25% decrease for butylhydroxytoluene and green tea, respectively (Figure 1).
The total EPR acquisition time for all three antioxidants and the control sample was around two hours, which shows the capability of EPR as a fast technique for evaluating the skin care product quality.
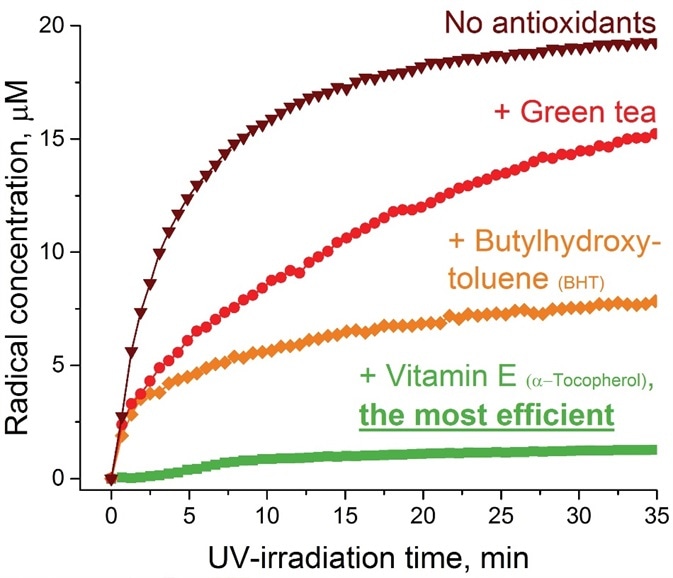
Figure 1. Effectiveness of antioxidants (green tea, BHT, and vitamin E) on skin care product aging upon UV-irradiation evaluated by EPR method.
EPR spectroscopy is also utilized successfully to monitor free radical formation throughout photodegradation of pharmaceutical products, pinpoint the radical intermediates, and help to establish the reaction mechanism.
Light can compromise drug product stability and may cause degradation of the inert materials (excipients), final medicinal products (formulations), or the active pharmaceutical ingredients (APIs), causing a loss of product potency or toxic by-product production.
Quite often, photodegradation processes require transition metals and free radicals which are responsible for the majority of the damage that happens in drug products. The International Conference of Harmonization (ICH) guidelines say that the intrinsic photostability characteristics of all new APIs and products must be assessed to establish that light exposure does not lead to unacceptable alteration.
For more than 20 years, these guidelines have been applied in the United States, Europe, and Japan. EPR spectroscopy can be utilized to learn the root cause of degradation, measure the extent of degradation, and anticipate long-term stability characteristics of the APIs, excipients and formulations.
The measurements are extremely fast and use just a small amount of the drug formulation or API because of EPR’s inherent high sensitivity, so the method could be employed during the early drug development phase2. For example, photodegradation of the hypertension drug Nifedipine after exposure to light exhibits the formation of a free radical (Figure 2).
EPR comes after the time evolution of the free radicals, and the signal is recognized as N-based free radical formed because of the photodegradation of the API. The growing number of radicals measured by EPR exhibit the level of API degradation and can be utilized to estimate the product photostability.
Free radical photochemical reactions are extremely common in beverages and food, they also play a key role in food photodegradation. Additionally, there is strong evidence for the huge part free radicals play in numerous diseases. Yet, there are also studies exhibiting that free radicals produced during food processing may add to flavor formation, texturization, and other reactions involving characteristic qualities and food properties.
So, distinguishing and understanding photochemical reactions which are free radical-mediated in beverages and foods, and learning to control these reactions concerning shelf-life stability and food qualities gives good reason to be interested in EPR identifying and examining radicals in foods and beverages.
At the start of this article the effects of UV-light on beer associated with beer ‘skunking’ was mentioned. Another example of food photodegradation is rancidity of edible oils. It occurs during transport or storage and is a result of the free-radical mediated photooxidation of unsaturated fatty acids that influences quality and in many cases, decides the shelf-life of the oil.
Fatty acid photodegradation happens in three well-documented stages:
- Initiation
- Propagation
- Termination
The resulting oxidized compounds at the end of the process give the oil an undesirable taste and odor. Using EPR stress testing, oxidation profiles and accurate calculation of free radical concentrations supply a measure for oxidative resistance at every part of the production process (Figure 3).
This allows manufacturers to make quick and informed process control choices to increase product shelf-life. EPR spectroscopy has been applied successfully in examinations of photodegradation reactions in material science involving products like fuel cells, solar cells, batteries, etc.
Paramagnetic imperfections like vacancies, defects, and free radicals influence the performance and properties of these materials as a result. So, it is critical to identify and characterize those species. Utilizing the EPR technique, one can gain insight into the stability ‘soft spots’ of the materials which is crucial for the development of robust products.
Susarova et al. for example, employed EPR as a highly sensitive analytical method for quality assessment of conjugated polymers and their performance in organic solar cells (3). It has been demonstrated that different batches of the same polymer have different photovoltaic performances correlating with the respective free radical concentrations. EPR data exhibits that this is due to structural defects or impurities which have unpaired electrons (Figure 4).
It is believed that these free radicals act as deep traps for mobile charge carriers, consequently influencing the performance of the conjugated polymers in solar cells. Photodegradation can be a two-edged sword. Photodegradation is growing in importance in the area of wastewater treatment, particularly for wastewater which contains small amounts of refractory organic substances, for example pharmaceutical residues.
A large number of pharmaceuticals have been discovered in drinking and surface water globally, which shows their ineffective removal from water and wastewater by utilizing conventional treatment technologies. Concerns have been raised over the possible detrimental effects of pharmaceuticals on public health and aquatic environments.
Amid the different treatment choices, photochemical advanced oxidation processes (light AOPs) are favorable for the efficient degradation of pharmaceuticals and other organic pollutants in water and wastewater.
Usually, AOPs are based on the chemistry of hydroxyl radicals (•OH), which are non-selective reactive oxygen species that can oxidize water pollutants into inactivated end-products.
Examples of light AOPs include:
- Photo-Fenton chemistry
- Ozone/UV-radiation
- Heterogeneous photodegradation using TiO2 as a photocatalyst
- H2O2/UV-radiation
Articles exist which compare different AOPs using their effectiveness to extract the organic pollutants, even assessed by cumulative concentrations such as total organic carbon and natural organic matter.
Yet, the •OH concentration, and therefore the oxidation efficiency, depends strictly on the AOP conditions so that comparison of the technique performance is not related to a single variable, but to a large number of parameters which do not permit a rationalization of the results.
The only method to detect, identify, and quantify hydroxyl radicals generated in light AOPs is EPR and so it can be employed to develop design and optimization of the AOP of interest to acquire the most effective treatment and decrease operating costs(4).
Photocatalysis Studies Using EPR
Low cost, non-toxicity, stability, and appropriate photocatalytic activeness are examples of the criteria for a good photocatalyst. Titanium dioxide meets these criteria for industrial-scale use and is predisposed to a huge scope of applications in numerous areas (self-cleaning surfaces, gas sensors, photocatalysts, thin film capacitors, solar cells, etc.)
The photoactivity of TiO2 is typically established by the process of electron/hole pair generation, recombination, interfacial transfer, and by the surface reactions of the charge carriers with the species on the surface of the photocatalyst adsorbed.
TiO2 acquires its activity from when photons of a particular wavelength are incident upon its surface, electrons are promoted from the valence band and transferred to the conductance band. This reaction creates positive holes in the valence band, which in turn react with the hydroxylated surface to create •OH radicals, which are the most potent oxidizing agents, as discussed above (Figure 5).
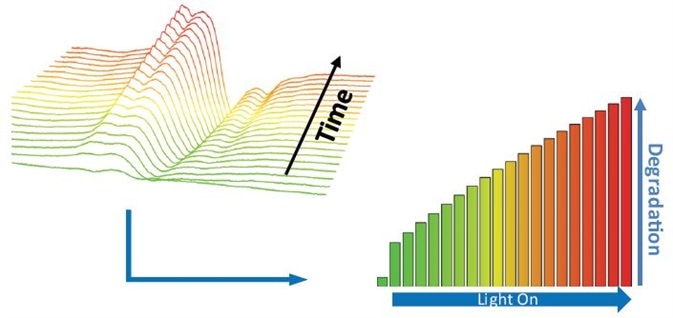
Figure 2. Photodegradation of Nifedipine – time evolution of N-based radical formed in the API and monitored by EPR.
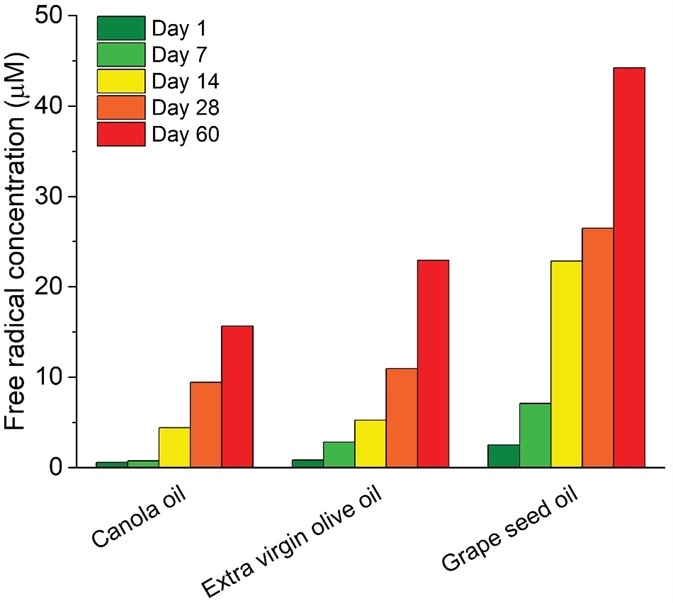
Figure 3. Quantitative EPR radical analysis after window exposure of edible oils for 60 days.
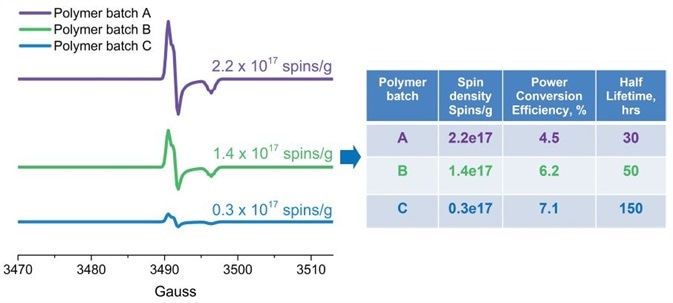
Figure 4. Quantitative EPR analysis of free radical defects presented as spins/g in conjugated polymers (used in solar cells). The number of defects correlates with the solar cell efficiency and stability.
Figure 5. Scheme of photocatalysis (taken from Ref. (5)).
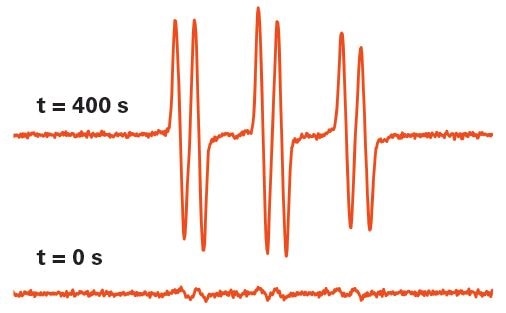
Figure 6. Hydroxyl radical detection by EPR in UV-irradiated TiO2 after 0 and 400 sec. The spin trapping technique was used to capture the radicals.
A further EPR application in photocatalysis involves the detection of paramagnetic reactive oxygen species (ROS) throughout photocatalytic activities of nanomaterials. For instance, Vankayala et al. described that singlet oxygen is produced via photo-irradiation of gold nanoparticles which can be utilized as photothermal agents for treating cancerous tumors (6).
A number of micro- and nanomaterials can initiate ROS production under numerous experimental conditions which can be seen as an intrinsic parameter of a particular nanomaterial, like other physiochemical characteristics like morphology, particle size, etc.
EPR is employed to develop suitable ROS-active nanomaterials for particular applications since their photobiological and photocatalytic activity corresponds with the ROS concentration monitored by the resonance method (7).
Study of Photopolymerization Using EPR
Modern technologies are always on the lookout for new and efficient radical photoinitiators, as free radical photopolymerization is the most frequently utilized application so far. Resulting from the absorption of light, a photoinitiator induces free radical chemistry reactions that instigate significant alterations in the solubility and physical characteristics of suitable formulations.
EPR spectroscopy is utilized to identify and monitor free radical intermediates, with a spotlight on the underlying mechanisms. For instance, EPR methods were applied successfully in the development of an innovative type of silyl radical producing photoinitiators employed for photopolymerization of methacrylates, e.g., in dental materials (8). As seen in Figure 7, three different radical intermediates were detected and identified by EPR.
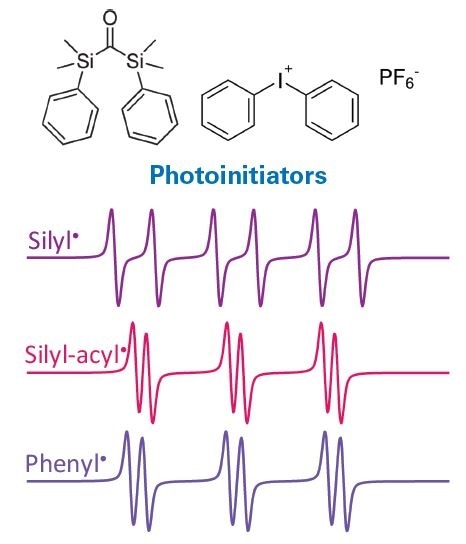
Figure 7. EPR spectra of radical species generated in photoinitiator bis-silylketone used for photopolymerization of methacrylates, e.g., in dental materials.
Additionally, quantitative EPR analysis supplied information about the photopolymerization efficiency and radical reactivity. The data was seen to be thought of as an initial idea for the development of other photoinitiating systems in this series.
To summarize, understanding photochemical reactions is critical for a number of applications in technology and science. EPR supplies the unique ability to directly measure the radicals that play a role in these reactions.
EPR is the solution for better understanding free radical photochemistry reactions by detecting, identifying, monitoring, and quantifying radical intermediates, and so helps to enhance products’ performance and their shelf-life.
References
- Gerlock J.L. et al., Determination of active HALS in automotive paint systems II: HALS distribution in weathered clearcoat/basecoat paint systems, Polym. Degrad. Stab. (2001) 73 201
- Williams H. et. al., Predicting the photostability characteristics of active pharmaceutical ingredients using electron paramagnetic resonance spectroscopy, Drug Dev. Ind. Pharm. (2012) 38(2) 200
- Susarova D. et al., ESR spectroscopy as a powerful tool for probing the quality of conjugated polymers designed for photovoltaic applications, Chem Commun. (2015) 51 2239
- Brame J. et al., Trading oxidation power for efficiency: Differential inhibition of photo-generated hydroxyl radicals versus singlet oxygen, Water Res. (2014) 60 259
- Saracino M. et al., Water remediation 2.0: Advanced oxidation processes, La Chimica & l’Industria, October (2015)
- Vankayala R. et al., Morphology dependent photosensitization and formation of singlet oxygen (1Δg) by gold and silver nanoparticles and its application in cancer treatment, J. Mater. Chem. B (2013) 1 4379
- He W. et al., Composition directed generation of reactive oxygen species in irradiated mixed metal sulfides correlated with their photocatalytic activities, ACS Appl. Mater. Interfaces (2015) 7 16440
- Graff B. et al., Development of novel photoinitiators as substitutes of camphorquinone for the LED induced polymerization of methacrylates: a bis-silyl ketone, Macromol. Rapid Commun. (2017) 38 1600470
About Bruker BioSpin - NMR, EPR and Imaging
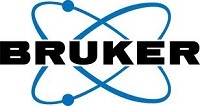
Bruker BioSpin offers the world's most comprehensive range of NMR and EPR spectroscopy and preclinical research tools. Bruker BioSpin develops, manufactures and supplies technology to research establishments, commercial enterprises and multi-national corporations across countless industries and fields of expertise.
Sponsored Content Policy: News-Medical.net publishes articles and related content that may be derived from sources where we have existing commercial relationships, provided such content adds value to the core editorial ethos of News-Medical.Net which is to educate and inform site visitors interested in medical research, science, medical devices and treatments.