Introduction
The Main Players: Types of Brain Cells
The Mechanics of Neural Communication
Non-Neuronal Communication
How Brain Communication Shapes Thought and Behavior
When Communication Breaks Down
Cutting-Edge Research and Technologies
Related Video
References
Illustration of neurons within the brain. Image Credit: 3Dme Creative Studio / Shutterstock
Introduction
The human brain contains nearly 86 billion neurons, constantly exchanging messages like an immense social media network, but neurons do not work alone – glial cells, neurotransmitters, receptors, and other molecules form a vast communication web that governs everything from thought to behavior. Inside each neuron, electrical impulses travel rapidly, while communication between neurons takes place across microscopic gaps called synapses. At these junctions, neurotransmitters like dopamine, glutamate, or serotonin act as chemical messengers, binding to receptors on neighboring cells to influence activity. Supporting this system are receptor types such as ligand-gated ion channels (LGICs) and G-protein–coupled receptors (GPCRs), each triggering distinct responses. Alongside these, neurotrophins such as brain-derived neurotrophic factor (BDNF) and hormones shape long-term brain function and development.1
This article explores how the brain's neurons and supporting glial cells communicate through electrical and chemical signals, shaping everything from thought to behavior, and how disruptions in this network contribute to neurological disorders.
Summary of the roles accomplished by glial cells in the brain discussed in this review. Each glial cell, microglia, astrocyte, and oligodendrocyte, achieves crucial roles in the healthy developing and mature brain. Colors were used to highlight the similar roles between glial cells2.
The Main Players: Types of Brain Cells
The brain is composed of a complex network of cells, with neurons and glial cells being the principal players in maintaining communication and function. Neurons are the primary signaling units, transmitting electrical and chemical messages through specialized structures: dendrites (which receive signals), axons (which transmit them), and synapses (where neurons connect and communicate). However, this intricate messaging system is supported and modulated by glial cells – astrocytes, oligodendrocytes, and microglia.2
Astrocytes maintain the chemical environment, regulate blood flow, and support synaptic activity. Oligodendrocytes insulate axons by forming myelin sheaths, allowing rapid signal conduction. Microglia act as immune sentinels, pruning synapses and clearing debris. These glial cells are not mere support elements; emerging research highlights their dynamic role in modulating neural circuits, influencing learning, memory, and mood. Microglia and astrocytes, for instance, directly interact with synapses in “quad-partite” models of communication, indicating a deeper integration into neuronal signaling than previously thought.2
Other non-neuronal cell types, such as NG2-glia and ependymal cells, also contribute to brain homeostasis and signaling, though they are less well characterized.
In essence, brain communication is a collaborative endeavor. Neurons send messages, but glial cells shape, sustain, and sometimes even initiate these conversations, revealing that understanding brain function requires studying both neuronal and non-neuronal cells in tandem.2
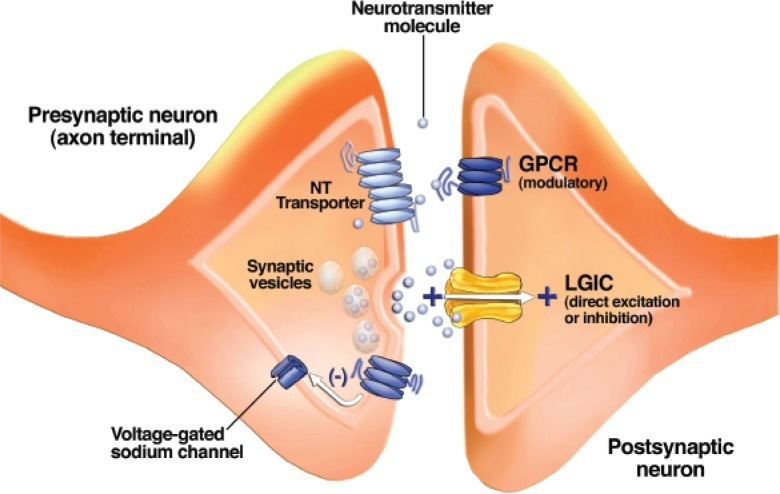
Schematic drawing of a synapse between two neurons. Synaptic vesicles contain a neurotransmitter (NT) and release it when their membranes fuse with the outer cell membrane. Neurotransmitter molecules cross the synaptic cleft and bind to receptors known as ligand-gated ion channels (LGICs) and G-protein–coupled receptors (GPCRs) on the postsynaptic neuron. GPCRs on the presynaptic neuron’s axon terminal alter the function of voltage-gated ion channels and modulate neurotransmitter release. Neurotransmitter transporters remove neurotransmitter molecules from the synaptic cleft so that they can be repackaged into vesicles1.
The Mechanics of Neural Communication
Neural communication relies on rapid electrical and chemical signaling. Within a neuron, charged particles like sodium and potassium ions flow through specialized proteins called ion channels, generating electrical impulses. The most critical of these is the action potential – a brief voltage spike that travels along the axon to the synapse.
At the synapse, neurons communicate chemically. When an action potential reaches the axon terminal, it triggers calcium influx, prompting vesicles to release neurotransmitters into the synaptic cleft. These chemical messengers such as dopamine, serotonin, and glutamate cross the gap and bind to receptors on the receiving (postsynaptic) neuron.
LGICs create fast responses by allowing ion flow that excites or inhibits the postsynaptic neuron. Excitatory synapses promote action potentials by depolarizing the membrane, usually via sodium influx. Inhibitory synapses, often through chloride channels, hyperpolarize the membrane, reducing the chance of signal transmission.1
GPCRs trigger slower but longer-lasting intracellular cascades affecting cell activity, gene expression, and neurotransmitter release. Their effects are subtler and often termed neuromodulatory. For example, dopamine often acts via GPCRs such as D1 and D2 receptors to modulate mood, attention, and movement. Together, this blend of electrical and chemical communication allows the brain’s 86 billion neurons to form dynamic networks. Disruption in these processes caused by disease, drugs, or injury can impair mood, memory, and behavior. Understanding these mechanics is key to developing treatments for neurological and psychiatric disorders.1
Non-Neuronal Communication
Astrocytes play active roles in non-neuronal communication by modulating both synaptic function and cerebral blood flow. In the concept of the “tripartite synapse,” astrocytes form close physical and functional associations with presynaptic and postsynaptic neurons. These cells express receptors that detect neurotransmitters such as glutamate and acetylcholine, which allows them to respond to neuronal activity. In response, astrocytes can release gliotransmitters like glutamate, adenosine triphosphate (ATP), and D-serine, influencing synaptic strength and plasticity. They also regulate neurotransmitter clearance and recycle them back to neurons in precursor forms, maintaining synaptic homeostasis.3
Beyond synaptic communication, astrocytes play a critical role in neurovascular coupling. Their end-feet envelop blood vessels, allowing them to sense neuronal activity and respond by releasing vasoactive substances like prostaglandin E₂ and epoxyeicosatrienoic acids. These substances dilate blood vessels, adjusting cerebral blood flow according to local metabolic demands. Astrocytes also contribute to the structural integrity of the blood-brain barrier and regulate ion and water homeostasis through channels and transporters. Thus, astrocytes are integral not only to neuron-glia signaling but also to coordinating neural activity with vascular response.3 However, some aspects of gliotransmitter release mechanisms remain debated in the scientific community.
How Brain Communication Shapes Thought and Behavior
The brain communicates through intricate networks of neurons that continuously rewire themselves – a process known as neural plasticity. Every time we learn something new or form a memory, neurons adjust their connections, strengthening or weakening synapses based on activity. This dynamic rewiring forms the foundation for learning, adaptation, and even recovery after injury. Alongside structural changes, electrical patterns known as brain waves help coordinate activity across distant brain regions. These oscillations occur at different frequencies, such as alpha, beta, or gamma waves, and play crucial roles in attention, sleep, and decision-making. Fast waves often indicate alertness, while slower ones are linked to relaxation or deep sleep. Together, these patterns help integrate sensory input, internal states, and motor responses.4
Scientists hypothesize that consciousness itself may emerge from this coordinated brain-wide activity. Theories suggest that when different brain regions synchronize, especially through high-frequency waves, they create a unified, conscious experience. In essence, our thoughts, memories, and behaviors arise from the brain’s ability to wire, fire, and harmonize across regions. Understanding these communication mechanisms not only deepens insight into how we think and feel but also holds promise for treating conditions like epilepsy, depression, and neurodegenerative disorders.5
When Communication Breaks Down
When neural communication fails, the brain’s delicate balance is disrupted, leading to neurological disorders such as Parkinson’s disease, epilepsy, schizophrenia, and Alzheimer’s disease. These conditions often originate from faulty signaling at synapses, where neurotransmitters like dopamine, glutamate, and γ-aminobutyric acid (GABA) regulate brain activity. In Parkinson’s disease, dopamine-producing neurons degenerate, impairing movement coordination. In schizophrenia, dopamine signaling becomes excessive or misdirected, contributing to hallucinations and disorganized thoughts. Epilepsy arises from uncontrolled bursts of electrical activity due to imbalances between excitatory and inhibitory synapses. Alzheimer’s disease involves widespread neuronal death, often linked to neuroinflammation and malfunctioning synapses.1
Common mechanisms behind these disorders include abnormal levels of neurotransmitters (e.g., too much or too little dopamine), autoimmune attacks on synaptic proteins, and reactive gliosis – a state in which glial cells overreact to injury, promoting inflammation rather than repair. This glial response can impair neurovascular coupling and hinder neuron-glia communication, compounding damage. Moreover, neuroinflammation and disrupted neurotransmitter removal exacerbate signal breakdown, leading to progressive cognitive and behavioral decline. Understanding these breakdowns in synaptic and cellular communication is essential for developing therapies aimed at restoring proper brain function in these debilitating disorders.1
Cutting-Edge Research and Technologies
Recent advancements in neuroscience have led to transformative tools that unlock how the brain communicates and controls behavior. Brain-Computer Interfaces (BCIs) stand at the forefront, enabling direct communication between neural activity and external devices. By decoding brain signals, BCIs allow patients with neurological disorders like stroke or Parkinson’s disease to control prosthetics, wheelchairs, or computer systems using thought alone. The integration of artificial intelligence enhances signal decoding, enabling highly personalized rehabilitation through adaptive feedback systems.6
Equally transformative is optogenetics and real-time imaging, which let researchers precisely control and visualize neural circuits in living brains. This allows unprecedented insight into how specific neurons influence behavior, emotion, and learning. Through techniques like functional magnetic resonance imaging (fMRI) and optogenetic stimulation, scientists can observe communication pathways and synaptic changes in action.6
Meanwhile, connectomics and AI-powered simulations are mapping entire neural networks at synapse-level detail. Using electron microscopy and machine learning, researchers have reconstructed full connectomes in model organisms like the fruit fly, whose relatively simple nervous systems make them ideal for this purpose. These maps reveal how neurons organize into circuits that process sensory inputs, drive decisions, and coordinate movement. Importantly, computational models based on these maps now simulate neural activity and behavior, helping decode how structure translates into function.6
Together, these technologies not only deepen our understanding of the brain but also pave the way for revolutionary treatments – from thought-controlled mobility aids to brain-based therapies for mental illness and cognitive decline.
Related Video
Beautiful 3-D Brain Scans Show Every Synapse | National Geographic
References
- Lovinger, D. M. (2008). Communication networks in the brain: neurons, receptors, neurotransmitters, and alcohol. Alcohol Research & Health, 31(3), 196. https://pmc.ncbi.nlm.nih.gov/articles/PMC3860493/
- Carrier, M., Dolhan, K., Bobotis, B. C., Desjardins, M., & Tremblay, M. È. (2022). The implication of a diversity of non-neuronal cells in disorders affecting brain networks. Frontiers in Cellular Neuroscience, 16, 1015556. https://www.frontiersin.org/journals/cellular-neuroscience/articles/10.3389/fncel.2022.1015556/full
- Liu, C. Y., Yang, Y., Ju, W. N., Wang, X., & Zhang, H. L. (2018). Emerging roles of astrocytes in neuro-vascular unit and the tripartite synapse with emphasis on reactive gliosis in the context of Alzheimer’s disease. Frontiers in Cellular Neuroscience, 12, 193. DOI:10.3389/fncel.2018.00193, https://www.frontiersin.org/journals/cellular-neuroscience/articles/10.3389/fncel.2018.00193/full
- Nayak, M., Das, D., Pradhan, J., Ahmed, R. G., Laureano-Melo, R., & Dandapat, J. (2022). Epigenetic signature in neural plasticity: the journey so far and journey ahead. Heliyon, 8(12). DOI: 10.1016/j.heliyon.2022.e12292, https://www.cell.com/heliyon/fulltext/S2405-8440(22)03580-0
- Tam Hunt, UC, Jonathan Schooler, University of California Santa Barbara. The easy part of the Hard Problem: A resonance theory of consciousness. Frontiers in Human Neuroscience. https://www.frontiersin.org/journals/human-neuroscience/articles/10.3389/fnhum.2019.00378/full
- Zhang, H., Jiao, L., Yang, S., Li, H., Jiang, X., Feng, J., Zou, S., Xu, Q., Gu, J., Wang, X. and Wei, B. (2024). Brain–computer interfaces: the innovative key to unlocking neurological conditions. International Journal of Surgery, 110(9), 5745–5762. DOI: 10.1097/JS9.0000000000002022, https://journals.lww.com/international-journal-of-surgery/pages/articleviewer.aspx?year=2024&issue=09000&article=00043&type=Fulltext