The major inhibitory amino acid transmitter within the mammalian central nervous system (CNS) is GABA. Largely all of the brain’s neurons respond to GABA, while approximately 20% use it as their primary transmitter.1
Using iontophoretic application of GABA to CNS neuronal preparations, primary electrophysiological studies demonstrated that it produced inhibitory hyperpolarizing responses2 that were blocked competitively by the alkaloid bicuculline.3 In the late 1970s, Bowery et al, were attempting to identify GABA receptors on peripheral nerve terminals. They observed that GABA application lessened the evoked release of noradrenalin within the heart of the rat, and that this effect was not blocked by bicuculline. Baclofen, a compound unable to produce rapid hyperpolarizing responses in central neurons, mimicked this action of GABA. To differentiate it from the more familiar receptor type which had become known as GABAA, this newly identified receptor was named GABAB.4,5 Using the conformationally restricted GABA analog, CACA6,7, another bicuculline-insensitive receptor was also identified. On the recommendation of the IUPHAR Nomenclature Committee, this receptor GABAA–ρ (previously named GABAC) has now been subsumed into the GABAA receptor class.8
The GABAA Receptor
Distribution and Function
Within the CNS, GABAA receptors are widely but differentially distributed. Several GABA isosteres can activate these receptors, including muscimol and isoguvacine10. Some of these ligands proved significant, after radiolabeling, in the early delineation of receptor distribution. Receptor activation results in an increased membrane chloride conductance,11,12 which typically causes an influx of Cl– and membrane hyperpolarization. Positive cooperativity is generally exhibited by concentration-response curves, which is consistent with the presence of a minimum of two agonist binding sites on each receptor molecule.13–15
The agonist-induced current decreases as a consequence of receptor desensitization, on continued exposure to high agonist concentrations.16–18 The first estimates of mean single-channel conductance and average channel open times was provided by biophysical characterization of the receptor, though initially carried out using noise analysis of neurons in primary culture.19 The average channel open times varied with the nature of the activating agonist.20 Further detail was provided on the nature of single channel events with the development of single channel recording techniques21 , which demonstrated multiple single channel conductances: 44, 30, 19 and 12pS,22 the 30pS conductance being the most widespread.
Concentration of the compound determines both channel opening times and opening frequency; bicuculline, a competitive antagonist, modulates both of these parameters and reduces the conductance.23,24 There are other competitive antagonists, including the pyridazinyl GABA derivative, SR 95531. The receptor can also be blocked non-competitively by a number of bicyclohosphates and picrotoxin.25 Additionally, penicillin decreases channel open probability in a way which is compatible with open channel block.26
Receptor Diversity
In the 1980s, purification of the bovine brain receptor revealed two major subunits of the GABAA receptor, which were respectively named α and β. Subunit-specific monoclonal antibodies were raised with elucidation of partial amino acid sequences of these subunits, which allowed exploration of the fine anatomical detail of receptor distribution.27 The sequence data also facilitated the cloning of the first two GABAA receptor subunit isoforms.28,29
A multiplicity of protein subunits was revealed by subsequent molecular studies revealed a multiplicity of protein subunits, which have now been divided into seven classes. These classes were based upon the extent of similarities in their deduced amino acid sequences, and within each there are further subdivisions into subunit isoforms, some of which exhibit alternate splice variants. Presently, in humans six α-, three β-, three γ- and three ρ- subunit isoforms are known, with single representatives of the δ, ε, π and θ classes. The sequence homology is approximately 70% within a single subunit class; however, between classes this falls to around 30%. In other species, additional isoforms of some of these classes are known.30 The three ρ-subunits were considered to define the GABAC receptor in earlier receptor nomenclature.8
Homologies and a common structural organization were revealed by deduced amino acid sequences from each of the subunits, which places them firmly within the so-named Cys-loop ligand-gated ion channel (LGIC) family. These receptors are pentamers of homologous subunits, which link to create a central ion channel traversing the cell membrane. The peripheral nicotinic acetylcholine receptor (nAChR) is the archetypal member of the family, with other members of the family including glycine and 5-HT3 receptors.
Each of the homogolous subunits possesses a long amino terminal domain of over 200 amino acids, which carries the signature cys-cys loop. Four hydrophobic segments follow this extracellular domain, each of which is approximately 20 amino acids long. These four segments are named TM1–TM4, and were predicted to form transmembrane domains with TM2 contributing to the generation of the ion channel lining. There is a large intracellular loop between TM3 and TM4, which is the most dissimilar part of the sequence within the GABAA receptor subfamily.
Evidence currently suggests that only a limited number of GABAA receptor subunit combinations are expressed in vivo, despite the plethora of receptor subunits.31 Each subunit is encoded by a separate gene and by combining in situ hybridization and immunohistochemical studies, it has been revealed that there is a distinct distribution of the various gene products in the CNS.32,33 This finding corroborates the concept that each receptor subtype is comprised of combinations of subunits and serves defined physiological roles. This, in turn, offers significant findings regarding the development of subtype-selective pharmaceutical agents. Nonetheless, there is an added complication in that the expression patterns of individual subunits are not immutable, and can change throughout their development, both in response to normal physiological cycles and also as a consequence of pharmacological intervention.34–37
In the mammalian CNS, most receptors are comprised of α-, β- and γ-subunits, with the most common receptor subtype containing the α1, β2 and γ2 isoforms.38 Using recombinant receptors expressed in mammalian cells or Xenopus ooctyes, the functional characteristics of individual GABAA receptor subtypes have been extensively explored. To determine the roles of individual subunits, peptide segments and specific amino acids in receptor function, numerous mutagenesis studies have been carried out. It is apparent that it is essential to have an accurate view of the overall receptor structure if we are to interpret mutagenic results effectively at the molecular level.
Structure and Function
When the sequence homologies of many subunits of the Cys-loop LGIC family were first revealed, it seemed reasonable to anticipate that all members would share the same structural organization as the Torpedo nAChR. This, as the best-characterized member of the family, has been imaged using cryoelectron microscopy (most recently at a resolution of 4Å).39 It is a pentamer of homologous subunits that are ordered pseudosymmetrically around an integral ion channel. A pentameric structure of similar diameter (about 8 nm across the pentamer) was revealed by using electron microscopy to image the purified porcine GABAA receptor by negative staining.40
It is currently believed that the most abundant α1β2γ2 GABAA receptor subtype is made up of two copies each of the α1- and β2-subunits together with a single γ2- subunit.41 Concatenation, an approach that involves physical linking of the cDNAs encoding two or more subunits before their ectopic expression with other subunits, was first use to study the arrangement of the subunits within the pentamer.42 This, and other similar studies, has demonstrated a pentameric subunit arrangement of β-α-βα-γ in an anticlockwise direction, when viewed from the outside of the cell.43
It has been possible to use the 4Å structure of the Torpedo nAChR as a template to construct in silico models of this most common GABAA receptor subtype with this information44,45 (Figures 1a and 1b). Thus, a means is provided through this modeling, by which one can explore the similarities and differences in the structure and function of different members of the GABAA receptor subfamily.
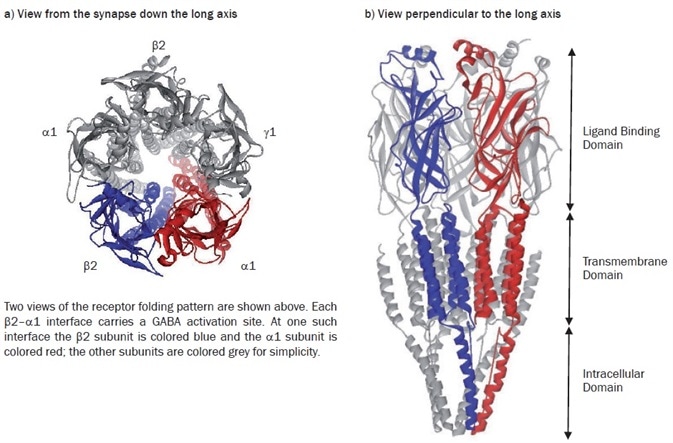
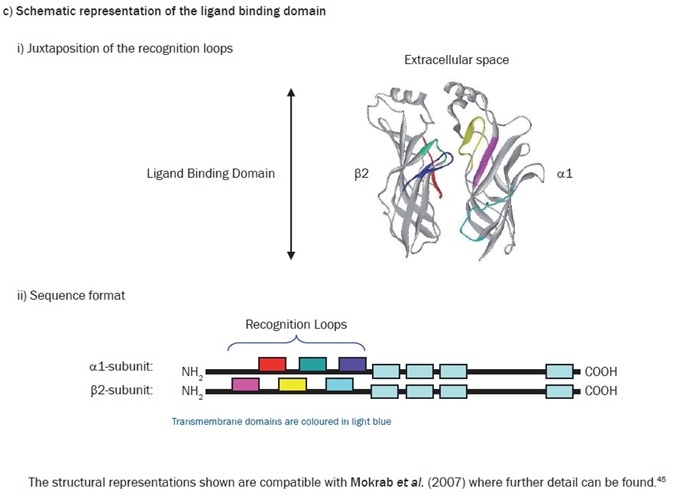
Figure 1. Model of the α1β2γ2 GABAA receptor structure. Image credit: Tocris Bioscience
For four decades, the analysis and study of ligand recognition in the extracellular domains of the Cys-loop family of receptors has been continuing. A somewhat unexpected source provided renewed interest in this in 2001. The structure of a water-soluble acetylcholine binding protein (AChBP) from Lymnaea stagnalis was determined at 2.7Å resolution.46 This structure soon proved to be a valuable homolog of the extracellular segment of the nAChR, and other members of the family. It was, in fact, the first protein to be used as a template with which to model the extracellular domain of the α1β2γ2 GABAA receptor.47 This model furnished the first direct structural evidence that corroborated the long-standing idea that GABA recognition sites were located at the β-α interfaces, with the plethora of mutagenic data available in the literature at the time. It additionally rationalized much hitherto experimental data which indicated that an allosteric site for the classical benzodiazepines occupies a similar position at the adjacent α-γ interface.
When considering the GABA activation sites, the current consensus is that the primary determinants of agonist recognition are located within at least six non-contiguous stretches (‘loops’) of amino acids in the extracellular domains of each subunit, where loops A–C are being contributed by the ‘principal’ subunit (β) and loops D–F contributed by the neighboring ‘subordinate’ subunit. Some homology is revealed by sequence comparisons of the ‘recognition loops’ in divergent subunits of the receptor superfamily. However, the family members are differentiated by the structural divergence within these loops, which provides the exquisite acuity of ligand recognition. The value of this in silico approach has proved to be significant. In addition to allowing visualization of the location of amino acids involved in ligand recognition, by using theoretical ligand docking approaches, it also becomes plausible to address receptor subtype-selective ligand design, which is an area of huge and undeniable commercial interest.48
Given that the binding sites for both neurotransmitters and allosteric modulators occur at subunit-subunit interfaces, the importance of the subunit arrangement within each pentameric receptor must again be considered. Indeed, as stated, there is much theoretical and experimental evidence which indicates that the subunit arrangement of the α1β2γ2 receptor is secure. Nonetheless, there is no a priori reason to decide that less abundant receptors adopt a comparable pentameric architecture. For example, what is the arrangement of subunits in receptor subtypes which consist of αβδ subunits?
Recently, atomic force microscopy (AFM) was recently used to address this question and investigate the α4β3δ subtype.49 The subunits were C-terminally tagged with different epitopes and the receptor-antibody complexes were visualized by AFM after ectopic expression and detection with the appropriate antibodies. A similar arrangement to the α1β2γ2 subtype was suggested by the results, with the δ-subunit simply replacing the γ-subunit within the pentamer. Despite this, we cannot exclude the possibility of heterogeneity in receptor assembly, and, for example, results from concatenation studies suggest that the δ-subunit may be a little more prolific than first suggested.50
Both from a physiological and a pharmacological perspective, comparison of the two receptor subtypes described above (α1β2γ2 and α4β3δ) is important. It has become clear throughout the past few years that there are two major types of GABAA receptor-mediated inhibitory responses, which we term either phasic or tonic.51 Activation of GABAA receptors that are localized primarily to the synapse such as the abundant α1β2γ2 subtype results in phasic inhibition. Tonic transmission, however, is mediated by less abundant extrasynaptic receptors, which includes the α4β3δ subtype. These receptors are thought to be activated by the low concentrations of the natural agonist that escape the efficient re-uptake machinery found in both neurons and glia.
Developing drugs that have differential effects on these two forms of inhibitory neurotransmission is currently seeing a resurgence of interest. Though the natural agonist, GABA, appears to be a full agonist at the α1β2γ2 receptor, THIP (also known as gaboxadol), its conformationally restricted analog, is a partial agonist. Contrastingly, THIP is a full agonist at the α4β3δ receptor where GABA acts as a partial agonist. The hypnotic properties exhibited by THIP 52 are, interestingly, functionally very distinct from those of the most widely used hypnotics (namely zopiclone and the α1-selective agents, zolpidem and zaleplon). By interacting with classical benzodiazepine-sensitive receptors at the synapse, the latter compounds facilitate phasic inhibition. THIP appears to modulate tonic inhibition mediated by extrasynaptic receptors to produce its effects,53 which may also be selective targets for general anesthetics.
Modulators of GABAA Receptor Function
The Benzodiazepines
A significant impetus to GABAA receptor research is the therapeutic importance of the benzodiazepines. Agonist-mediated activation of the GABAA receptor is potentiated by classical benzodiazepines, which cause a parallel leftward shift of the GABA concentration-response curve. The discovery of saturable, high-affinity binding sites for [3H]-diazepam in the brain54,55 in 1976 provided their study with an important experimental tool.
All the overt effects of the benzodiazepines are mediated by GABAA receptors: sedative, anxiolytic, anticonvulsant, muscle relaxant and amnestic. However, benzodiazepines are not recognized by all the GABAA receptors. The primary determinant of benzodiazepine recognition is a particular α-subunit isoform present within an individual GABAA receptor subtype. The receptor fails to recognize the classical benzodiazepines if the α1-subunit is replaced by α4 or α6. From both biochemical and mutational analysis, it is now clear that this insensitivity can be attributed to a single amino acid substitution in the extracellular N-terminal domain: a histidine (H101) in the α1-, α2-, α3- and α5-subunits is replaced by an arginine residue in α4 and α6.56,57
All are recognized by the classical benzodiazepines when receptors containing the former subunits are expressed with a β- and γ2-subunit. However, we must note that several agents differentiate between the subtypes using the basis of a particular α-subunit isoform present in the pentamer. Triazolopyridazine CL 21887258 was the first to be identified, which is related to the recently introduced hypnotic, zaleplon. Similarly, β-carboline-3-carboxylic acid esters also possess a preference to certain α-subunit-containing receptors.59 Zolpidem is currently the most widely prescribed hypnotic in the USA, and has been shown to have high affinity for α1-containing receptors, lower affinity for receptors carrying α2 or α3 and very low affinity for those containing α560,61, with no observable interaction with receptors which contain the α4- or α6-subunits.
The importance of the α-subunit histidine-arginine substitution has been turned into an advantage using knockin (KI) technology. The switch’s exquisite specificity dictates that, through the act of replacing the α1 histidine with an arginine (H101R) in the germ line, the KI adult animals will only diverge from their wild counterparts in the ability of their α1-containing receptors to recognize the benzodiazepines. It was therefore expected that characterization of the knockin mouse phenotype would permit dissection of the complicated pharmacological effects of benzodiazepines based on their interactions with specific GABAA receptor subtypes.
This approach and its extensions have proven immensely valuable; now it is apparent that the α1-subunit is responsible for the sedative, the anterograde amnestic and certain anticonvulsant effects of the benzodiazepines,62,63 whereas the α2-subunit has been associated with their anxiolytic actions.64 Some of the results are less clear-cut; for instance, the pharmacodynamic profile of the α3 selective ligand, TP003, implies that this subunit contributes to both anxiolytic and anticonvulsant effects.65,66 In addition, receptors containing the α5-subunit have been indicated as contributors in learning and memory processes.67 Using conditional knockin studies has recently advanced this approach and revealed selective changes in the ability of GABAA receptors within particular cell groups to recognize the aforementioned hypnotic, zolpidem.68 Regrettably, attempts made to isolate the functional importance of individual GABAA receptor subunits using gene knockout technology have proved frustrating. Clearly, ablation of subunit expression results frequently in compensatory changes in the expression of other subunits, which creates notable obstacles in the task of assigning specific responsibility for the resulting phenotype.38
The development of the inverse agonist concept is perhaps one of the most interesting phenomenological observations to arise from studies of benzodiazepine interactions with the GABAA receptors. In this, certain non-benzodiazepine ligands were discovered that could displace a radiolabeled benzodiazepine from its binding sites. Among the first of these was ethyl β-carboline-3-carboxylate (β-CCE), which was shown to have diametrically opposed effects to those produced by classical benzodiazepines – for instance, it is proconvulsant. This led to a new terminology; β-CCE became known as an inverse agonist, and the classical benzodiazepines were then classified as agonists.69,70 In vitro electrophysiological experiments using inverse agonists demonstrated that they shift the GABA concentration response curve to the right, decreasing the natural transmitter’s potency. Therefore, while the agonist benzodiazepine site ligands increase channel opening frequency, this is decreased by the inverse agonists.71
Within the β-carboline series, the full efficacy spectrum is found: the ethyl ester is proconvulsant and thereby acts as a partial inverse agonist, the propyl ester is largely devoid of efficacy and is therefore termed an antagonist,72 while aromatic substitution in the A ring produces agonists which have similar properties to the classical benzodiazepines73. The pharmaceutical industry has not ignored the therapeutic potential afforded by the inverse agonist concept, with the work on partial inverse agonists selective for α5-containing receptor subtypes as cognition enhancers.74
Steroids
The first observation for allosteric steroid sites on these receptors was the discovery that 5α-pregnan-3α-ol-11,20-dione (alphaxalone), a synthetic steroidal anesthetic, could enhance stimulation-evoked inhibition produced by GABAA receptor agonists in rat cuneate nucleus slices75. Stereoselective activity of the progesterone metabolites 5α-pregnan-3α-ol-20-one (allopregnanolone), 5β-pregnan-3α-ol20-one (pregnanolone) and 5α-pregnan-3α,21-diol-20-one (allotetrahydrodeoxycorticosterone was confirmed by subsequent voltage clamp studies conducted on both neurons and adrenomedullary chromaffin cells.76,77
Mechanistically, the action of these compounds held apparent similarities to the barbiturates, which at low concentrations potentiate the effects of GABA by the increase of channel open times and, at higher concentrations, directly activating the receptor.78–82 It was revealed by later studies that the sites of barbiturate and steroid action are separate and distinct from one another.83 Conserved residues within the α- and β-subunit membrane spanning domains of the α1β2γ2 receptor have been identified, and are important for both steroid facilitation and direct activation.84
Studies with ectopically expressed receptors comprising αβγ subunits displayed the limited impact of subunit composition on the functional effects of the steroids.85 There is evidence, at putative extrasynaptic receptors, where δ replaces the γ-subunit, for increased steroid potency.86 Nonetheless, we may explain these observations, at least in part, by the reduced efficacy of the endogenous neurotransmitter, GABA, at these receptor subtypes.87 Myriad literature reports demonstrate that the potency of steroids differs between brain regions, as well as indicating that the observed effects may be influenced by changes in receptor phosphorylation and modulation of enzymatic activity in the steroid metabolic pathways.88 Normal physiological fluctuations in steroid levels associated with, for example, pregnancy and the ovarian cycle, amplify this functional complexity yet further. These can cause patterns of subunit expression which may contribute to the mood swings associated with these processes.89,37
General Anesthetics
Undeniably, we may now state that GABAA receptors play a significant role in general anesthesia. A large portion of the receptor subtypes are sensitive to clinically relevant concentrations of general anesthetics and exhibit the appropriate stereospecificity. These agents have diffuse characteristics; they exhibit sedative, hypnotic, analgesic and amnestic properties as well as causing a loss of mobility.90 This multitude of effects, as well as their structural diversity, renders it complex and difficult to dissect the actions of general anesthetics at the molecular level. One such agent, ketamine, chiefly affects glutamatergic excitatory responses mediated by NMDA receptors, and there is a dearth of evidence that the older anesthetics, nitrous oxide and xenon, modulate GABAergic inhibition.
Proof continues to grow regarding interactions of other inhalational and intravenous agents with the GABAA receptors. Given that general anesthetics are hydrophobic and need to access the CNS, it is perhaps predictable that they target hydrophobic pockets within the receptor’s transmembrane domains. Primary evidence implies that the inhalational anesthetics favor α-subunits91, while in vitro and in vivo evidence has grown to indicate that intravenous anesthetics interact with the β-subunits.92,93
The past decade of research has clarified that significant effects of general anesthetics occur as a result of their effects on extrasynaptically located receptors and not by their ability to potentiate the fast phasic inhibition mediated by synaptically located receptors. It seems clear that the extrasynaptic α5β3γ2 receptor in the hippocampus is likely associated with the amnestic actions of many of these agents.94 Indeed, the receptors containing δ-subunits in the ventrobasal thalamic nucleus offer an interesting link to the reversible loss of consciousness in humankind, a sleep-related phenomenon that is a primary characteristically incited by general anesthetics.95 The diversity of agents and targets offer key information that must be addressed systematically to maximize the potential for development of novel anesthetic agents.96
Alcohol
Much speculation abounds on the receptors responsible for the pharmacological effects of ethanol; however, there are only a small number of putative targets which are responsive to low concentrations of ethanol (<20 mM). Primary observations of altered ethanol-induced behaviors in δ-subunit knockout mice97 preceded in vitro studies of recombinant GABAA receptors. Significant academic excitement ensued when it was reported that agonist activation of δ-containing GABAA receptors could be facilitated by 10 mM ethanol;98,99 however, it has proved not possible to replicate replication of this response, and many of these discrepancies remain unexplained.100 Suggestions have arisen that many of the in vivo effects of ethanol could be attributed to indirect effects arising from its ability to heighten levels of several endogenous steroids which can in turn potentiate GABAA receptor-mediated responses. Supportive evidence for this theory arises from observations that, as well the correlation between consequential effects of ethanol and an increase in steroid levels, they are also inhibited by blockers of steroid synthesis.101
Phosphorylation may here be significant, given that it has been noted that in PKCδ knockout mice, the pharmacological effects of ethanol are lessened, as are the ataxic responses to both pentobarbital and pregnanolone. As the animals’ flunitrazepam response remained intact, the suggestion arose that the overt effects were mediated by benzodiazepine insensitive GABAA receptors. Additional studies demonstrated that the PKCδ-dependent effects of ethanol could be seen in ectopically expressed α4β3δ receptors.102 Therefore, it remains difficult to assign individual effects of ethanol to specific GABAA receptors.
GABAA-ρ Receptors (Previously GABAC)
It is useful to highlight the distinguishing features of the receptors originally designated as GABAC, even though they are now considered to be members of the GABAA family.8 The receptors were originally classified thanks to their unique pharmacology. GABA, the natural agonist, was reported to be about an order of magnitude more potent at this subclass than at other GABAA receptors. Although CACA activated this receptor, this agent remained unrecognized by either the GABAA or GABAB classes. GABAA-ρ receptor responses were not inhibited by bicuculline but, much like the GABAA receptors, picrotoxin blocked them. Later, a selective GABAA-ρ receptor antagonist, (1,2,5,6-tetrahydropyridine-4-yl) methylphosphinic acid7 (TPMPA) was identified.
Additional pharmacological differences from the GABAA receptors were identified, which included its lack of modulation by the barbiturates, benzodiazepines, or neuroactive steroids. Receptors that showed these characteristics were demonstrated to have a restricted distribution, which was initially found in the spinal cord and subsequently the retina6,103, the latter of which being the source from which the first ρ-subunit was cloned.104 Now, three homologous ρ-subunits, ρ1 to ρ3, have been identified. These can be depicted as either homomers or heteromers105,106 and the ectopically expressed receptors portray the pharmacological characteristics of GABAA-ρ receptors.
There is very little evidence that the ρ-subunits co-assemble with any of the other GABAA receptor subunits107. The genes encoding the ρ1- and ρ2-subunits are found on chromosome 6 in humans, and therefore are distinct from the clusters of GABAA receptor subunit genes to be are found on chromosomes 4, 5, 15 and X (with the exception of δ, which is found on chromosome 1). The ρ-subunit sequences show a figure between 30 and 38% homology to the GABAA receptor subunits at the amino acid level, however they show greater homology to the glycine α-subunits than to any of the GABAA receptor subunits in the important TM2 region of the sequence.
The GABAB Receptor
The additional key class of GABA receptors is the metabotropic (G-protein-coupled) GABAB receptor. A distinct ligand recognition profile to the GABAA receptor family is exhibited,4 and these are differentially found within the mammalian CNS.108 Functionally, they inhibit adenylyl cyclase activity109 and presynaptic calcium channels, which decreases transmitter release,110 and activates postsynaptic potassium channels, as well as producing the late inhibitory postsynaptic potential.111
Distribution and Function
The seminal evidence to distinguish the GABAB receptor from more familiar members of the GABAA receptor family was provided by the initial observation that GABA inhibited the release of noradrenalin from rat atria in vitro, an effect not blocked by bicuculline methobromide but mimicked by (R)-baclofen.4 Subsequent analyses which relied upon both functional and radioligand binding techniques, managed to further refine the structure-activity profile at GABAB receptors112. Though the receptor is commonly distributed within the mammalian CNS, it is generally located at lower densities than GABAA receptors, and displays a distinct distribution. That is to say, the highest concentrations are found in the molecular layer of the cerebellum, the frontal cortex and certain thalamic nuclei.108 The receptor is additionally to be found in the periphery, wherein its activation modulates autonomic intestinal control and decreases esophageal reflux.112,113
The receptor is paired to adenylyl cyclase via Gi and Go proteins. Activation of presynaptic GABAB receptors (though the consequences remain poorly defined) also leads to the inhibition of high voltage-activated Ca2+ channels, an effect mediated by the G protein βγ subunits. Decreased transmitter release results, and potentially also limitation of synaptic vesicle recruitment to the active zone.114 GABA activation of postsynaptic GABAB receptors causes hyperpolarization through the modulation of inwardly rectifying KIR3-type K+ channels115 that mediate the late phase of the inhibitory postsynaptic potential.
Molecular Characterization
In 1997, the molecular characterization of the GABAB receptor was achieved, when the availability of specific high-affinity antagonists permitted the expression cloning of the GABAB1 subunit.116 Subsequently, it was demonstrated through various studies that while this protein displayed lots of the expected characteristics (when expressed ectopically) it paired poorly to its effector machinery, and displayed a remarkably low affinity for agonists compared to the native receptor. It appeared, too, to be retained within the endoplasmic reticulum.117 Subsequently, studies found the identity of an additional subunit, GABAB2,118–120, which interacted with the GABAB1 subunit C-terminus, masking the ER retention signal of the GABAB1 subunit121 and assisting the trafficking of the GABAB1 subunit to the cell surface. This evidence provided the first secure proof of receptor dimerization.
Class C of the GPCRs contains the GABAB receptor, with the metabotropic glutamate receptors mGlu1–8 and the calcium-sensing receptor.122 Each subunit has a large N-terminal extracellular domain exhibiting the Venus fly-trap motif, which is followed by 7-transmembrane helices as well as an intracellular C-terminus. We know two splice variants exist for the GABAB1 subunit: these are encoded by the same gene and arise by alternate promoter usage to produce GABAB1a and GABAB1b.123 They differ only in their N-terminal domains. GABAB1a possesses a repeat of a conserved protein-binding motif, which are termed ‘sushi domains’, and which are lacking in GABAB1b; the first 147 amino acids of GABAB1a are replaced by 18 amino acids in GABAB1b.124
Though both subunits that are contained in the GABAB heterodimer display the Venus fly-trap motif at the extracellular N-terminus, it is the GABAB1 subunit that is the principal force of agonist and antagonist recognition (the residues responsible are not conserved in the GABAB2 subunit).125 There is also a serine residue within this recognition domain that seems to be responsible for the receptor’s ability to sense Ca2+ concentrations.126
The presence of the GABAB2 subunit markedly increases the agonist affinity of the GABAB1 subunit, though it is not primarily responsible for agonist recognition.127 The GABAB2 subunit mediates G protein-coupling, with the second intracellular loop as particularly important,128,129 though clearly GABAB1 is significant in the facilitation of this process. The GABAB2 subunit seems to be the interaction site for an increasing family of positive allosteric modulators130, where binding occurs within the transmembrane domain131 to augment agonist tone though it exhibits no direct agonist activity.132
A significant frustration has been the restricted molecular heterogeneity found in the GABAB receptor population, given that ectopic expression studies have not provided support for the varied functional responses ascribed to these receptors in vivo.133 Knockout studies which target GABAB1 or GABAB2 have not managed to alleviate these difficulties, with both deletions producing similar phenotypes, although each compromises the expression of the conjugate subunit.134,135 Functional distinctions have begun to emerge between the GABAB1 subunit isoforms, which indicate that the GABAB1a isoform is largely associated with the heteroceptors that control the release of glutamate.136–139
Suggestions have arisen that this differential cellular localization may be associated with the presence of the sushi repeats, which are present in GABAB1a but not in GABAB1b, and which are known to be important in protein-protein interactions in other environments.140 Recently, interesting developments have indicated that a soluble truncated form of the GABAB1a subunit, termed GABAB1j, displays nanomolar affinity for neuronal membranes. This is indistinguishable from the first 157 amino acids of the GABAB1a subunit and contains the sushi repeats, as well as a 72-amino acid C-terminal extension with no homology to other known proteins. Both basal and stimulated glutamate release are decreased in its presence, but GABAB receptor function at presynaptic autoreceptors or postsynaptic receptors continues to be unaffected.141
Clinical Potential
The only clinically available agent that targets the GABAB receptor is Baclofen. Introduced into clinical practice in 1972, long before the discovery of the GABAB receptor, it continues to be the favorite intervention to address the spasticity associated with cerebral palsy and multiple sclerosis. Baclofen has a challenging side effect profile on systemic administration, as it is responsible for causing drowsiness, nausea, muscle weakness and mental confusion. These are largely due to the poor brain penetration necessitating the use of high oral doses.142 The muscle relaxant effects, which are mediated within the spinal cord, can be secured by intrathecal administration, permitting a dose reduction and limiting both the systemic effects development of tolerance.143 Antinociceptive properties at the spinal level are also exhibited by Baclofen, which again permits local administration,144 however, significant analgesia is also mediated within the ventrobasal nucleus of the thalamus,145 (a site which requires systemic administration). Today, the analgesic effects of baclofen have a limited use in humans.
Early specific GABAB receptor antagonists had a limited potency, with phaclofen, as an example, displaying an affinity of only 100 μM. Several, high-affinity and systemically active antagonists are now available, which have vast clinical potential in absence epilepsy.142 Mice which exhibit characteristics associated with atypical absence epilepsy overexpress the GABAB1a isoform.147 Contrastingly, recent reports suggest that impaired GABAB receptor function may be a possible contributor in repetitive firing in human temporal lobe epilepsy tissue.148 An open trial was the first clinical exploration of GABAB receptor antagonists, with SGS 742 (CGP 36742) in mild cognitive deficit.149 Although the initial results appeared promising, clinical developments have not reached the canon of literature. Recently, studies suggested that mechanistically phospho-protein kinase A (pPKA) has a key role to play in the effects of this antagonist in the Morris water maze.150
For some time, it has been known that the GABAB receptor activation effectively reduces the craving for addictive drugs. This was primarily exhibited as a reduction in cocaine self-administration in rats,151 and with other addictive drugs of abuse similar findings have emerged.142 It may be that positive allosteric modulators at the receptor may be a preferable means of control. These agents could reasonably be expected to facilitate GABAB receptor-mediated tone which would circumvent the side-effect profile associated with the use of systemic agonists. Studies have also recently suggested that compounds of this type hugely reduce cocaine self-administration in rats152,153, with similar approaches providing some support for their potential as anxiolytics.154
The GABAB receptors remain somewhat clinically mysterious, as promissory notes of significant therapeutic potential are yet to materialize significantly. In addition, it has been argued that the dearth of differentiable receptor subtypes has restricted the opportunity for discrete drug targeting. Recent studies continue to expand the evidence for their functional importance, as they highlight their impact on both the tegmental pedunculopontine nucleus, which is important in the acute rewarding effects of the opiates155 and orexin neurons, associated with sleep/wakefulness cycles.156
Clinical applications continue to be enigmatic though allosteric modulators may prove to be as valuable in the modulation of tonic activity at the GABAB receptors, as the benzodiazepines are within the GABAA receptor family. The development of novel GABAB receptor agonists is currently under investigation, particularly the structure thereof which effectively restricts them to the peripheral compartment. Research is being undertaken regarding their potential intervention in gastroesophageal reflux in man, after proving efficacious and crucially devoid of the baclofen-associated central side effects in preclinical studies.157
Conclusions
It is the ionotropic receptor for the major inhibitory neurotransmitter GABA that has achieved the most visibility to date, despite the overwhelming representation of the GPCRs in the human genome. A plethora of intriguing insights into the mechanisms by which communications within the nervous system are achieved and the nuances of modulation provide opportunities for further refinement of pharmacological intervention, thanks to its serendipitous exploitation within the clinical arena.
The lack of molecular heterogeneity exhibited by the GABAB receptors has proved problematic for specific drug targeting. The functional characterization in the mammalian system of this receptor has been predicted to lead to significant clinical developments. Though this goal remains to be seen, recent research provides new and exciting opportunities for therapeutic intervention. The next decade of research will certainly prove to be exciting.
Special Mentions
This white paper was based on the original work of:
All three authors share common interests in GABAergic transmission
Ian L. Martin: Professor of Pharmacology in the School of Life and Health Sciences, Aston University, Birmingham, UK
Norman G. Bowery: Emeritus Professor of Pharmacology, University of Birmingham, UK
Susan M.J. Dunn: Professor and Chair at the Department of Pharmacology, Faculty of Medicine and Dentistry, University of Alberta, Canada. Email: [email protected]
References
- Somogyi et al. (1998) Brain Res.Rev. 26 113.
- Krnjevic and Schwartz (1967) Exp.Brain Res. 3 320.
- Curtis et al. (1970) Nature 226 1222.
- Bowery et al. (1980) Nature 283 92.
- Hill and Bowery (1981) Nature 290 149.
- Johnston et al. (1975) J.Neurochem. 24 157.
- Johnston (1996) TiPS 17 319.
- Barnard et al. (1998) Pharmacol.Rev. 65 68-76.
- Palacios et al. (1981) Brain Res. 222 285.
- Ebert et al. (1997) Mol.Pharmacol. 52 1150.
- Curtis et al. (1968) Exp.Brain Res. 5 1.
- Kelly et al. (1969) Exp.Brain Res. 7 11.
- Dichter (1980) Brain.Res. 190 111.
- Sakmann et al. (1983) J.Neural Transm. 18 83.
- Bormann and Clapham (1985) Proc.Natl.Acad.Sci.USA 82 2168.
- Adams and Brown (1975) J.Physiol. 250 85.
- Akaike et al. (1987) J.Physiol. 391 219.
- Mathers (1987) Synapse 1 96.
- Barker et al. (1982) J.Physiol. 322 365.
- Barker and Mathers (1981) Science 212 358.
- Hamill et al. (1981) Pflugers Arch. 391 85.
- Bormann et al. (1987) J.Physiol. 385 243.
- Macdonald et al. (1989) J.Physiol. 410 479.
- Twyman et al. (1990) J.Physiol. 423 193.
- Bowery et al. (1976) Br.J.Pharmacol. 57 435.
- Twyman et al. (1992) J.Physiol. 445 97.
- Richards et al. (1987) J.Neurosci. 7 1866.
- Stephenson and Barnard (1986) in Benzodiazepine/GABA chloride channels: structural and functional properties. p261 Alan R Liss.
- Schofield et al. (1987) Nature 328 221.
- Hevers and Luddens (1998) Mol.Neurobiol. 18 35.
- Olsen and Sieghart (2008) Pharmacol.Rev. 60 243.
- Wisden et al. (1992) J.Neurosci. 12 1040.
- Pirker et al. (2000) Neuroscience 101 815.
- Laurie (1992) J.Neurosci. 12 4151.
- Holt et al. (1996) Neuropharmacology 35 1457.
- Smith et al. (1998) J.Neurosci. 18 5275.
- Griffiths and Lovick (2005) Neuroscience 136 457.
- Rudolph and Mohler (2004) Ann.Rev.Pharmacol.Toxicol. 44 475.
- Unwin (2005) J.Mol.Biol. 346 967.
- Nayeem et al. (1994) J.Neurochem. 62 815.
- Farrar et al. (1999) J.Biol.Chem. 274 10100.
- Im (1995) J.Biol.Chem. 270 26063.
- Baumann (2002) J.Biol.Chem. 277 46020.
- Ernst et al. (2005) Mol.Pharmacol. 68 1291.
- Mokrab et al. (2007) J.Mol.Graph Model. 26 760.
- Brejc et al. (2001) Nature 411 269.
- Cromer et al. (2002) TiBS 27 280.
- Clayton et al. (2007) Curr.Med.Chem. 14 2755.
- Barrera et al. (2008) Mol.Pharmacol. 73 960.
- Kaur (2009) J.Biol.Chem. 284 7889.
- Farrant and Nusser (2005) Nature Neurosci.Rev. 6 215.
- Krogsgaard-Larson et al. (2004) Biochem.Pharmacol. 68 1573.
- Drasbek and Jensen (2006) Cereb.Cortex 16 1134.
- Squires and Braestrup (1976) Nature 266 732.
- Mohler and Okada (1977) Science 198 849.
- Wieland et al. (1992) J.Biol.Chem. 267 1426.
- Duncalfe et al. (1996) J.Biol.Chem. 271 9209.
- Squires et al. (1979) Pharmacol.Biochem.Behav. 10 825.
- Nielsen and Braestrup (1980) Nature 286 606.
- Hadingham et al. (1993) Mol.Pharmacol. 43 970. R
- Sanger et al. (1994) Neurosci.Behav.Rev. 18 355.
- Rudolph et al. (1999) Nature 401 796.
- McKernan et al. (2000) Nature Neurosci. 3 587.
- Low et al. (2000) Science 290 131.
- Dias et al. (2005) J.Neurosci. 25 10682.
- Fradley et al. (2007) J.Psychopharmacol. 21 384.
- Collinson et al. (2000) Eur.J.Neurosci. 12 171.
- Wulff et al. (2007) Nature Neurosci. 10 923.
- Tenen and Hirsch (1980) Nature 288 609.
- Cowan et al. (1981) Nature 290 54.
- Barker et al. (1984) in Actions and interactions of GABA and the benzodiazepines. p203 Publ Raven Press.
- Smiechen et al. (1993) in Anxiolytic β-carbolines: from molecular biology to the clinic. p7 Publ Springer Verlag.
- Stephens et al. (1987) Brain Res.Bull. 19 309.
- Sternfield et al. (2004) J.Med.Chem. 47 2176.
- Harrison and Simmonds (1984) Brain.Res. 323 287.
- Cottrell et al. (1987) Br.J.Pharmacol. 90 491.
- Harrison et al. (1987) J.Neurosci. 7 604.
- Study and Barker (1981) Proc.Natl.Acad.Sci.USA 78 7180.
- Twyman et al. (1989) Ann.Neurol. 25 213.
- Callachan et al. (1987) Neurosci.Letts. 29 S21.
- Nicoll et al. (1975) Nature 258 625.
- Simmonds (1981) Br.J.Pharmacol 73 739.
- Peters et al. (1988) Br.J.Pharmacol. 94 1257.
- Hosie et al. 2006 Nature 444 486.
- Belelli et al. (2006) Neuroscience 138 821.
- Brown et al. (2002) Br.J.Pharmacol. 136 965.
- Bianchi and MacDonald (2003) J.Neurosci. 23 10934.
- Herd et al. (2007) Pharmacol.Ther. 116 20.
- Koksma et al. (2003) J.Neurosci. 23 788.
- Bonin and Orser (2008) Pharmacol.Biochem.Behav. 90 105.
- Nishikawa et al. (2002) Neuropharmacology 42 337 .
- Belelli et al. (1997) Proc.Natl.Acad.Sci.USA 94 11031.
- Jurd et al. (2003) FASEB J. 17 250.
- Cheng et al. (2006) J.Neurosci. 26 3713.
- Franks (2008) Nature Neurosci.Rev. 9 370.
- Zeller et al. (2007) BMC Pharmacol. 7 2.
- Mihalek et al. (2001) Alcohol Clin.Exp.Res. 25 1708.
- Sundstrom-Poromaa (2002) Nature Neurosci. 5 721.
- Wallner et al. (2003) Proc.Natl.Acad.Sci.USA 100 15218.
- Borghese et al. (2006) J.Pharmacol.Exp.Ther. 316 1360.
- Morrow et al. (2001) Brain Res.Rev. 37 98.
- Choi et al. (2008) J.Neurosci. 28 11890.
- Polenzani et al. (1991) Proc.Natl.Acad.Sci.USA 88 4318.
- Cutting et al. (1991) Proc.Natl.Acad.Sci.USA 88 2673.
- Enz and Cutting (1998) Vision Res. 38 1431.
- Enz and Cutting (1999) Eur.J.Neurosci. 11 41.
- Sieghart & Ernst (2005) Curr.Med.Chem.Cent.Nerv.Sys. Agents 5 217.
- Bowery et al. (1987) Neuroscience 20 365.
- Hill (1985) Br.J.Pharmacol. 84 249.
- Dunlap and Fishbach (1981) J.Physiol. 317 519.
- Newberry and Nicoll (1985) J.Physiol. 360 161.
- Bowery et al. (2002) Pharmacol.Rev. 54 247.
- Ong and Kerr (1990) Life Sci. 46 1489.
- Sakaba and Neher (2003) Nature 424 775.
- Luscher et al. (1997) Neuron 19 687.
- Kaupmann et al. (1997) Nature 368 239.
- Couve et (1998) J.Biol.Chem. 273 26361.
- Jones et al. (1998) Nature 396 674.
- Kaupmann et al. (1998) Nature 396 683.
- White et al. (1998) Nature 396 679.
- Bettler et al. (2004) Physiol.Rev. 84 835.
- Pin et al. (2007) Pharmacol.Rev. 59 5.
- Steiger et al. (2004) J.Neurosci. 24 6115.
- Blein (2004) J.Biol.Chem. 279 48292.
- Kniazeff et al. (2002) J.Neurosci. 22 7352.
- Galvez et al. (2000) Mol.Pharmacol. 57 419.
- Galvez et al. (2001) EMBO J. 20 2152.
- Robbins et al. (2001) J.Neurosci. 21 8043.
- Duthey et al. (2002) J.Biol.Chem. 277 3236.
- Urwyler et al. (2001) Mol.Pharmacol. 60 963.
- Dupuis et al. (2006) Mol.Pharmacol. 70 2027.
- Pin et al. (2005) FEBS J. 272 2947.
- Ullrich and Bettler (2007) Curr.Opin.Neurobiol. 17 298.
- Schuler et al. (2001) Neuron 31 47.
- Gassmann et al. (2004) J.Neurosci. 24 6086.
- Vigot et al. (2006) Neuron 50 589.
- Perez-Garci et al. (2006) Neuron 50 603.
- Waldmeier et al. (2008) J.Neural Transm. 115 1401.
- Guetg et al. (2009) J.Neurosci. 29 1414.
- Grace et al. (2004) Proc.Natl.Acad.Sci.USA 101 12836.
- Tiao et al. (2008) J.Biol.Chem. 283 31005.
- Bowery (2006) Curr.Opin.Pharmacol. 6 37.
- Penn (1989) N.Engl.J.Med. 320 1517.
- Ataka et al. (2000) Pain 86 273.
- Potes et al. (2006) J.Neurosci.Res. 83 515.
- Pan et al. (2008) Pharmacol.Ther. 117 141.
- Wu et al. (2007) Neurobiol.Dis. 26 439.
- Teichgräber et al. (2009) Epilepsia 2009 50 1697.
- Froestl et al. (2004) Biochem.Pharmacol. 68 1479.
- Sunyer et al. (2009) Hippocampus 19 90.
- Roberts and Andrews (1997) Psychopharmacology 131 271.
- Smith et al. (2004) Psychopharmacology 173 105.
- Slattery et al. (2005) Neuropsychopharmacology 30 2065.
- Malherbe et al. (2008) Br.J.Pharmacol. 154 797.
- Heinmiller et al. (2009) Behav.Neurosci. 123 145.
- Matsuki et al. (2009) Proc.Natl.Acad.Sci.USA 106 4459.
- Alstermark et al. (2008) J.Med.Chem. 51 4315.
- Zhang et al. (2001) TiPS 22 121.
- Bowery and Enna (2000) J.Pharmacol.Exp.Ther. 292 2.
- Murata et al. (1996) Bioorg.Med.Chem.Lett. 6 2071.
- Ragozzino et al. (1996) Mol.Pharmacol. 50 1024.
- Sieghart (1995) Pharmacol.Rev. 47 181.
About Tocris Bioscience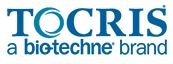
Tocris Bioscience is your trusted supplier of high-performance life science reagents, including receptor agonists & antagonists, enzyme inhibitors, ion channel modulators, fluorescent probes & dyes, and compound libraries. Our catalog consists of over 4,500 research tools, covering over 400 protein targets enabling you to investigate and modulate the activity of numerous signaling pathways and physiological processes.
We have been working with scientists for over 30 years to provide the life science community with research standards, as well as novel and innovative research tools. We understand the need for researchers to trust their research reagents, which is why we are committed to supplying our customers with the highest quality products available, so you can publish with confidence.
Tocris is part of the protein sciences division of Bio-Techne, which also includes the best in class brands R&D Systems, Novus Biologicals, ProteinSimple, and Advanced Cell Diagnostics. Bio-Techne has united these brands to provide researchers with a full portfolio of research reagents, assays, and protein platforms. For more information on Bio-Techne and its brands, please visit bio-techne.com.
Sponsored Content Policy: News-Medical.net publishes articles and related content that may be derived from sources where we have existing commercial relationships, provided such content adds value to the core editorial ethos of News-Medical.Net which is to educate and inform site visitors interested in medical research, science, medical devices, and treatments.